Skeletal Muscle and Tendon
Thomas M. Best MD, PhD, FACSM
John J. Wilson MD

Skeletal muscle has a complex organization of nerves, blood vessels, and connective tissue matrix that protects against injury and organizes individual cellular elements into functional contractile units of muscle.
Muscle fibers range a few millimeters to several centimeters in length. Fibers are arranged into larger units known as fascicles. Perimysium, a connective tissue sheath, surrounds fascicles. Whole muscles, made of numerous fasciculi, are surrounded by epimysium.
Tendon is juxtaposed between muscle and bone and is responsible for transmitting muscular forces to the skeletal system during limb locomotion.
The majority of skeletal muscle injuries that occur in sports are the result of indirect strain or direct blunt force trauma. Lacerations, ischemia, and infections are less common in athletics.
Indirect muscle strain injuries usually occur at the myotendinous junction during a stereotypical eccentric contraction. Certain muscles are more likely to sustain this type of injury (e.g., hamstrings during the rapid acceleration found in football, sprinting, and soccer).
Delayed muscle soreness (DMS) usually occurs at least 24 hours after exercise. Eccentric exercises, especially unfamiliar and repetitive ones, are more likely to predispose patients to DMS. Patients with DMS complain of pain, stiffness, and swelling in the muscles.
Direct trauma can occur at any point in the muscle. The quadriceps is the most common site of athletic muscle contusion, especially in football players. Under sufficient force, disruption of the muscle tissue and architecture can occur, often resulting in a hematoma.
Skeletal muscle accounts for nearly 45% of an average person’s body weight, making it the largest organ in the human body. Although it can be thought of as a single organ, skeletal muscle is highly compartmentalized into separate entities, each with its own unique role within a functional muscle. Muscle has a complex organization of nerves, blood vessels, and connective tissue matrix that protects against injury and organizes individual cellular elements into functional contractile units of muscle. Skeletal muscle generates forces that result in bodily movement, steady posture, joint stabilization, and heat production through normal contractile functioning. This chapter begins with a discussion of the cellular structure and architecture of muscle, including myofibrillar contractile proteins, and then proceeds to the larger subunits and, ultimately, to skeletal gross anatomy. A detailed knowledge of the muscle structure will aid in understanding the biomechanics and physiology of normal skeletal muscle described here as well.
Because muscles must exert their force on the human skeleton, the tendons that accomplish this task are discussed as well. Tendons are positioned between muscles and bones, so the tension generated by muscle contraction can be conveyed to the bone trigger locomotion. The myotendinous junction is the transition point between muscle and tendon, and the osteotendinous junction (OTJ) is the point of tendon insertion into bone. Like the muscles they serve, tendons have an intricate cellular structure of collagen fibrils, elastin proteins, extracellular ground substance, and fibroblast cells. Tendons also have a rich vascular supply and innervation, which are important for normal functioning. The biomechanical properties of tendons make them suitable for transmitting muscular forces to bone with minimal loss of energy or stretch.

Histology
Understanding the structure and architecture of skeletal muscle begins at the level of the muscle cell, or fiber, itself. Muscle fibers are multinucleated cells with a cylindrical shape, and they have diameters ranging between 10 to 100 μm. Fibers range a few millimeters to several centimeters in length. This wide variation in muscle fiber structure has important implications for their contractile mechanics. A covering of endomysium surrounds individual muscle fibers, and fibers are arranged into larger units, visible to the naked eye, known as fascicles. A connective tissue sheath known as the perimysium surrounds fascicles. Finally, whole muscles made of numerous fasciculi are surrounded by the epimysium (1) (Fig 4-1). The connective tissue surrounding muscle carries the vast array of blood vessels supplying the tissue with a rich vascular network. The membranes are intimately, but loosely, associated with muscle to allow the changes in length and diameter that occur with muscle contraction. The epimysium, perimysium, and endomysium also provide a broad attachment surface for muscles to join with tendons. The perimysium becomes continuous with the endotenon sheath of tendon at the myotendinous junction (2), and it allows the tendon to transmit the force that is generated by muscle contraction to result in limb locomotion. Tendons are considered more fully later in the chapter.
The arrangement of muscle fibers and fascicles in muscles varies, and this is apparent on gross inspection of muscle anatomy. Fibers can be arranged either parallel or at an angle to the longitudinal axis of muscle; the latter predominates and includes the unipennate, bipennate, fusiform, and multipennate types (Fig 4-2). The specific orientation of muscle fibers within a muscle affects a muscle’s physiological cross-sectional area (i.e., the estimated sum of the cross sectional areas of all the fibers).
Orientation of muscle fibers plays an important role in determining the contractile properties of a given muscle. Force production is proportional to the physiological cross sectional areas of muscles and fiber orientation, but the speed and absolute amount of shortening are proportional to the muscle fiber length. Shorter fibers have a lower velocity of shortening and a greater force production compared to longer fibers. Although a pennate arrangement of fibers results in a small reduction of the contractile force conducted to the tendon, pennate muscles have a greater number of fibers acting in parallel. Therefore, a pennate muscle will be more powerful (i.e., have a greater force of contraction) than a muscle of equal mass with a parallel arrangement. Contraction of the pennate muscle–tendon unit causes the tendon to move along the axis of force, increasing pennation of the muscle fibers.
Skeletal Muscle Cytology
Each muscle fiber is surrounded by its plasma membrane, or sarcolemma. Within this sarcolemma lie quiescent satellite cells that are essential for the repair of muscle after injury. Following muscle injury, inflammatory substances are thought to stimulate these satellite cells to undergo proliferation and differentiation into new muscle fibers (3,4). Fibers are further divided into smaller units called myofibers or myofibrils, which are made of sarcomeres arranged in series alignment. Sarcomeres are the basic unit of muscle contraction; they are made of myosin, actin, tropomyosin, and troponin proteins. Muscle fibers contain
a number of other intracellular organelles that are important for normal function as well. Each fiber has hundreds to thousands of nuclei that are found peripherally. Nuclei are the regulatory control centers of the cell, determining cellular material and distribution (e.g., synthesis). The amount and type of proteins to be synthesized is determined by the nuclear DNA, and it is carried out by ribosomes in response to mRNA encoding. Because of their ability to quickly up- and down-regulate protein synthesis, muscles are fairly adaptable. Depending on the type of muscle fiber, cellular mitochondria may account for nearly 20% of total cell volume (5), being most abundant in highly oxidative, slow-twitch fibers. Mitochondria are responsible for production of adenosine triphosphate (ATP) through oxidative metabolism. This ATP is the crucial energy source for skeletal muscle force production as well as most other cellular tasks. Muscle fibers also contain various amounts of enzymes, lipids, and glycogen.
a number of other intracellular organelles that are important for normal function as well. Each fiber has hundreds to thousands of nuclei that are found peripherally. Nuclei are the regulatory control centers of the cell, determining cellular material and distribution (e.g., synthesis). The amount and type of proteins to be synthesized is determined by the nuclear DNA, and it is carried out by ribosomes in response to mRNA encoding. Because of their ability to quickly up- and down-regulate protein synthesis, muscles are fairly adaptable. Depending on the type of muscle fiber, cellular mitochondria may account for nearly 20% of total cell volume (5), being most abundant in highly oxidative, slow-twitch fibers. Mitochondria are responsible for production of adenosine triphosphate (ATP) through oxidative metabolism. This ATP is the crucial energy source for skeletal muscle force production as well as most other cellular tasks. Muscle fibers also contain various amounts of enzymes, lipids, and glycogen.
Motor Unit
Muscle fibers are arranged into discrete entities known as motor units. The muscle fiber motor unit is the most basic neuromuscular contractile unit, and it is composed of a single α-motor neuron and all the muscle fibers that it innervates (6,7). A single motor neuron can innervate as few as 10, or as many as several thousand, muscle fibers (8). As a motor neuron enters its muscle at the motor point, the nerve axons branch many times, and each muscle fiber in the motor unit is contacted by a single nerve ending at the motor end plate (typically at the fiber midsection) (Fig 4-3). Adjacent muscle fibers do not typically belong to the same motor unit, because fibers from a single unit usually are distributed throughout the muscle (9). Also, fibers belonging to the same motor unit share similar fiber type and contractile characteristics (10,11). As few as 10 fibers may be seen per motor unit in areas such as ocular muscles, where fine motor control is necessary. In large, force-generating muscles such as the quadriceps, however, there may be thousands of fibers per motor unit (12).
Contraction of a motor unit is initiated by an action potential arising from the nerve cell body that lies in the anterior horn of the spinal cord. The action potential reaches the muscle at a synapse known as the motor end plate or neuromuscular junction. Nerve action potentials pass efficiently and rapidly from the spinal cord to peripheral muscle because of a myelin sheath surrounding the axon that serves as an electrical insulator. The myelin sheath surrounds peripheral nerves until they reach their destination with the motor end plate. At the motor end plate, the axon loses its myelin sheath and expands into a Schwann cell–enveloped synaptic terminal. The nerve terminal of the presynaptic axon forms many terminal folds that include thousands of synaptic vesicles containing the neurotransmitter acetylcholine (ACh). The ACh-containing vesicles congregate in specialized release sites called active zones, which are
adjacent to the postsynaptic folds of the muscle membrane known as the sole plate. The sole plate is a specialized region of the muscle sarcolemma that is characterized by numerous junctional folds containing ACh receptors and chemically gated ion channels. An action potential reaching the terminal axon will stimulate an influx of calcium ions through voltage-gated calcium channels into the presynaptic cell. The increased intracellular calcium concentration mediates the fusion of synaptic vesicles with the axon membrane and the release of ACh into the neuromuscular cleft. ACh diffuses across the cleft, then binds to the ACh receptors of the postsynaptic muscle membrane that mediate depolarization of the muscle sarcolemma, thereby initiating an action potential in the muscle. This action potential rapidly spreads along the muscle fiber to trigger calcium release and subsequent excitation–contraction coupling and, thereby, muscle contraction. ACh released by the axon terminal and bound to the muscular ACh receptors is rapidly deactivated by acetylcholinesterase enzymes in the synaptic cleft bound to the postsynaptic membrane. One byproduct of this enzymatic inactivation, choline, is then reabsorbed by the terminal axon and resynthesized to ACh for future transmitter release.
adjacent to the postsynaptic folds of the muscle membrane known as the sole plate. The sole plate is a specialized region of the muscle sarcolemma that is characterized by numerous junctional folds containing ACh receptors and chemically gated ion channels. An action potential reaching the terminal axon will stimulate an influx of calcium ions through voltage-gated calcium channels into the presynaptic cell. The increased intracellular calcium concentration mediates the fusion of synaptic vesicles with the axon membrane and the release of ACh into the neuromuscular cleft. ACh diffuses across the cleft, then binds to the ACh receptors of the postsynaptic muscle membrane that mediate depolarization of the muscle sarcolemma, thereby initiating an action potential in the muscle. This action potential rapidly spreads along the muscle fiber to trigger calcium release and subsequent excitation–contraction coupling and, thereby, muscle contraction. ACh released by the axon terminal and bound to the muscular ACh receptors is rapidly deactivated by acetylcholinesterase enzymes in the synaptic cleft bound to the postsynaptic membrane. One byproduct of this enzymatic inactivation, choline, is then reabsorbed by the terminal axon and resynthesized to ACh for future transmitter release.
Sarcoplasmic Reticulum
Activation of skeletal muscle contraction is triggered through the release of calcium ions from a system of membranous sacs within the cell known as the sarcoplasmic reticulum (SR). Release of calcium from the SR is accomplished through rapid propagation of the muscle action potential throughout the muscle fiber via a complex system of membranes. The muscle membrane system ensures that the muscle action potential initiated at the superficially located muscle end plate rapidly reaches the depths of the fiber and is then transmitted from one cell to the next with great velocity. The SR is made up of the longitudinal tubules and the transverse tubules (T tubules). The action potential is carried the length of the fiber’s surface by the longitudinal tubule and lateral sac system and to the inner fiber by T tubules. The T tubules penetrate the muscle fibers near the Z line perpendicular to the depths of the muscle fiber. Each T tubule is closely
associated with two lateral sacs, which in turn are connected with the longitudinal tubules. The two lateral sacs and their related T tubule are collectively known as a triad.
associated with two lateral sacs, which in turn are connected with the longitudinal tubules. The two lateral sacs and their related T tubule are collectively known as a triad.
The SR has a highly specialized membrane system for actively sequestering calcium and maintaining a low resting calcium concentration in the myoplasm. The maintenance of low myoplasmic calcium concentrations is accomplished by calcium adenosine triphosphatase (ATPase) pumps that transport calcium ions against a concentration gradient via cleavage of one ATP molecule. When a muscle action potential is transmitted along the surface of the sarcolemma and into the T tubule and longitudinal tubule system, the SR becomes more permeable to Ca2+, and Ca2+ is released from the SR into the myoplasm. Most of the free calcium binds to troponin to initiate contraction. Relaxation of the muscle occurs when the low myoplasmic Ca2+ concentration is restored via the Ca2+ ATPase pumps. This process is commonly referred to as excitation– contraction coupling.
Structural Proteins of Muscle
Myosin, actin, tropomyosin, and troponin are the four major structural proteins that make up muscle (Table 4-1). These proteins form the foundation of the basic contractile unit known as the sarcomere. The banding pattern seen in striated skeletal muscle comes from the repeating arrangement of two myofibrillar filaments, thick filaments and thin filaments.
Thick filaments are composed primarily of a large protein, myosin [∼470,000 Daltons (Da)]. Myosin accounts for approximately 55% of the total percentage of structural protein in muscle. The myosin molecule is hexameric, being composed of two heavy chains and four light chains. Trypsin can cleave the heavy chains to yield fragments of heavy meromysin (HMM), and light meromysin, (LMM) which account for the head and tail, respectively, of the myosin molecule (Fig 4-4). The HMM can be further divided into smaller fragments by papain cleavage to yield subfragments 1 and 2 (S1 and S2, respectively). The S1 fragment of HMM is responsible for the ATPase activity of myosin, allowing actin–myosin cross-bridging during muscle contraction. The thick filament also contains C protein, M protein, and titin. The C protein is involved in cross-bridge regions of the thick filament, and the M protein contains the creatine phosphokinase enzyme. Titin is an elastic protein that is thought to provide resistance to elongation of the sarcomere and to protect against overstretch (13).
Table 4-1 Relative Proportions of Myofibrillar Proteins in Rabbit Skeletal Muscle | ||||||||||||||||||||
---|---|---|---|---|---|---|---|---|---|---|---|---|---|---|---|---|---|---|---|---|
|
Thin filaments contain primarily actin, along with lesser amounts of tropomyosin and troponin proteins, which form an α-helical cylinder. The actin of thin filaments is anchored at the end of the sarcomere to the Z line. Thin filaments measure approximately 1.0 μm in length and are arranged in a hexagonal pattern around each thick filament (Fig 4-5). Actin thin filaments also are associated with troponin and tropomyosin proteins, which serve a regulatory function during contraction and are discussed later in this section. Nebulin is a cytoskeletal protein that has been proposed to regulate the length of the thin filament during contraction and stretch.
Myofibrils demonstrate a regular, repeating arrangement of dark and light bands every 2 to 3 μm when viewed with an electron microscope. The regular arrangement of striations in skeletal muscle results from the organization of thick and thin filament proteins within the sarcomere (Fig 4-6). The sarcomere extends from one Z band to another, with I bands and A bands in between. The Z band, or Z disk (from Zwishen-Schieben, which is German for “interim disk”), anchors thin filaments at either end of the sarcomere. The I (isotropic) band, which is made of actin, troponin, and tropomyosin, is less densely packed with proteins and, therefore, appears lighter under a polarizing microscope. The A (anisotropic) band is composed of myosin and the actin–tropomyosin complex and corresponds with the thick filaments. It is more densely packed with proteins, giving it a darker appearance when viewed with a polarizing microscope. In the center of the A band is a protein-dense band known as the H (Heller) zone that contains the center of the sarcomere, the M (Middle) line.
Sliding Filament Model of Contraction
The length of the thick and thin filaments remains constant during contraction, but the overall sarcomere length becomes shorter. The I band becomes shorter with contraction as the thick filaments slide past the thin filaments in a process known, appropriately, as the sliding filament model of muscle contraction. The sliding filament model was proposed by both Huxley and Simmons (14) and by Huxley (15) in 1971 and is still the guiding hypothesis for the molecular basis of muscle contraction.
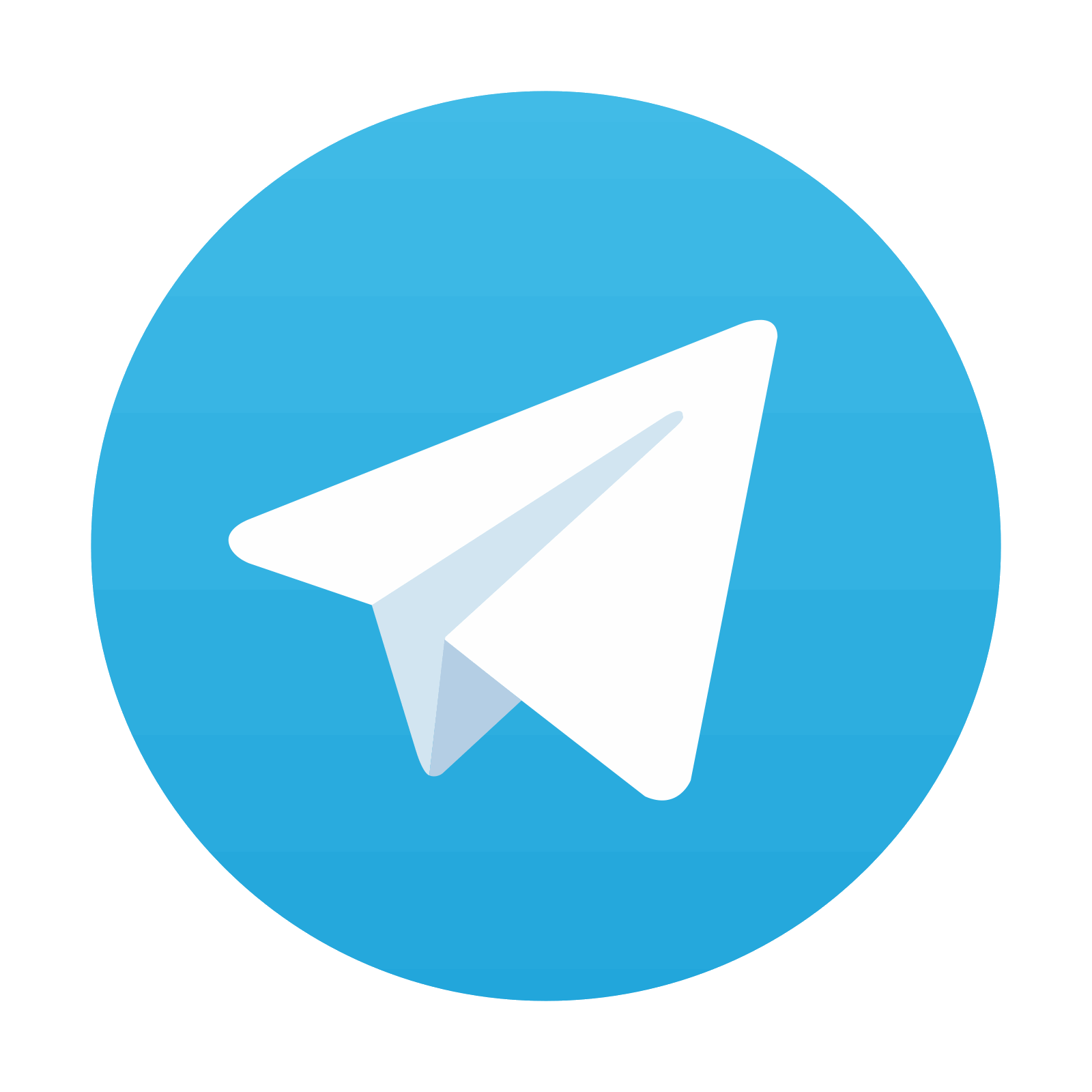
Stay updated, free articles. Join our Telegram channel
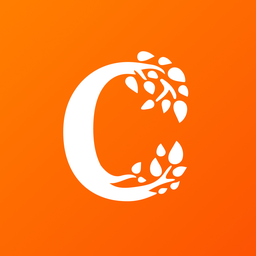
Full access? Get Clinical Tree
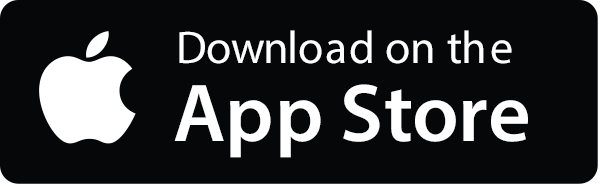
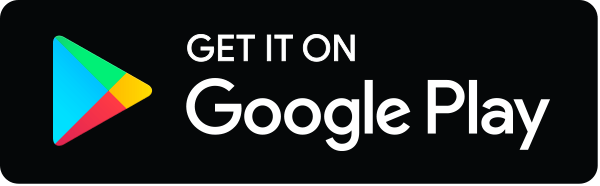