Theoretical whole-body heat loss (dry + evaporative) responses to rapid increases in metabolic heat production associated with exercise at fixed rates of heat production in the heat (30 °C/86 °F, 20% relative humidity). (From Wingo et al. [5], with permission)
Biophysical Determinants of Thermoregulation

where S is heat storage, M is metabolic heat production, W is work, K is conduction, R is radiation, C is convection, and E is evaporation. Positive S represents heat gain, and negative S represents heat loss. Internal body temperature will rise if heat gain exceeds heat loss (+S), and internal body temperature will fall if heat loss exceeds heat gain (–S). To maintain heat balance (S = 0), heat gain and heat loss must be equivalent. It should be noted that the heat exchange interaction between the environment and the body occurs across both the skin surface and respiratory tract. During exercise the magnitude of heat exchange across the skin surface is increased such that the skin factors can be considered the primary mechanisms of heat loss and gain from the body.
Metabolic Heat Production
Skeletal muscle metabolism yields two products, locomotion and heat. The amount of heat generated is directly proportional to the intensity of exercise with net heat gain being calculated by accounting for work (W) [6, 7]. Humans are relatively inefficient during exercise, with ~90% of energy being transformed to heat during treadmill exercise, yielding a mechanical efficiency of 10% [6, 7]. While this endothermy is necessary in many situations, during exercise in warm environments this heat can be excessive and lead to heat storage, resulting in increases in body temperature. The specific values for the heat production that results from metabolic energy expenditure can be calculated using calorimetry, which is estimated by rate of oxygen consumption [8]. Estimating metabolic heat production indicates how much heat must be lost to the environment to maintain heat balance and limit an increase in core temperature.
Radiation
Radiation is characterized as heat exchange in the form of electromagnetic waves between objects of different temperatures. Mean radiant temperature for the human body is dependent on the temperature of surrounding surfaces and changes with respect to the location of the heat source from the body [9]. This suggests that the orientation of the body from a radiant heat source, such as the sun, can greatly impact rate of radiant heat exchange. Some authors have suggested that ancestors of Homo sapiens adopted bipedalism to help mitigate thermal stress by reducing total body surface area exposed to the sun [10]. Limiting surface area exposure to the sun in other ways would also decrease heat gain via radiant heat but may inhibit other heat loss mechanisms.
Conduction
Conduction, as the direct transfer of heat through two solid objects in contact, generally plays a negligible role in exercise thermoregulation [11]. However, it should be noted that some cooling modalities, such as cooling vests, rely on conduction into a thermal medium for their cooling potential [12]. In the same fashion, direct contact with a heat source, more commonly seen in occupational settings (e.g., hot ovens, metal objects), can also contribute to heat gain.
Convection
Convective means of heating or cooling are the most intuitive aspects of human heat balance. For the purposes of human thermoregulation, we can consider our most common interaction with a fluid medium or air, as our primary convective interface [9]. In this sense, the gradient that dictates the rate of heat transfer is a function of the difference between skin temperature and ambient temperature [6]. As long as the temperature of the skin is greater than the surrounding air, heat loss can occur. This means that in most situations where ambient temperature is less than ~36 °C, convective cooling naturally occurs [13]. However, as soon as ambient temperature exceeds skin temperature, convective heating results in increasing thermal stress.
It is important to distinguish that convective heat exchange occurs on a local basis. Ambient temperature as reported by a weather station may not be representative of the local environment being experienced by an individual. Wet Bulb Globe Temperature (WBGT) reported by a weather station underestimates local athletic surfaces approximately 45% of the time [14]. A key consideration in this aspect is the concept of forced convection . In situations where there is no air movement, such as indoor exercise, convective cooling can decrease fairly rapidly due to the transfer of heat to the environment directly surrounding the individual [11]. Without air movement created by wind or a fan, the local temperature gradient between the skin and the environment can rapidly decrease.
This lack of forced convection is important to note in situations where individuals must exercise in enclosed environments. This most commonly manifests through the microenvironments created by protective equipment worn. While full body enclosures like nuclear, biological, and chemical warfare equipment fundamentally limit capacity for exercise in warm environments [15], even American football equipment covering some of the body surface can drastically increase heat stress [4].
Evaporation
Evaporative heat loss is a result of the eccrine sweating response. A sympathetic cholinergic activation of eccrine sweat glands results in sweat production on the skin surface for evaporation. Eccrine gland sweat secretion is an important avenue of heat dissipation as a result of the energy released through vaporization. For every gram of sweat evaporated from the body’s surface, the body loses 0.580 kcal or 2426 J of heat [16]. This results in a potent mechanism for dissipating heat in a variety of situations. In hot environmental conditions when ambient temperature exceeds skin temperature, evaporation is the only mechanism of heat loss to prevent further increases in body temperature. As a result, human thermoregulation is dependent on the appropriate dissipation of heat through evaporative cooling. Situations where evaporative cooling capacity is lower than the required demands of an environment and exercise combination are considered uncompensable heat stress, severely limiting the duration of time that an individual can perform a task.
Maximum evaporative heat loss (Emax) is dependent on the vapor pressure gradient between sweat on the skin surface and ambient air; which is a function of humidity, ambient temperature, and barometric pressure [6]. Unfortunately, when ambient temperature and relative humidity are high, the potential for evaporation is low, greatly diminishing the capacity of humans’ most powerful heat dissipation mechanism. In a simplified fashion, to maintain thermal equilibrium, Emax must be greater or equal to the evaporative requirements (Ereq) of the environment at a given metabolic rate. Exceeding the Ereq of the environment results in heat storage and uncompensable heat stress [17].
Emax is also dictated by skin wettedness (ϖmax) which is the proportion of total skin surface area of the body that is covered with sweat [18]. The more skin surface that is covered with sweat, the greater the Emax. Increased skin wettedness is acquired by recurrent exposure to increased thermal loads, which directly elicits alterations in sudomotor responses (typically occurring as part of heat acclimatization) [19]. ϖmax typically ranges from 0.85 to 1.0 (i.e., 85–100% body surface coverage of sweat). A heat-acclimatized individual is theoretically capable of attaining a ϖmax of 1.0, whereas non-heat-acclimatized individuals are considered to be unable to attain equivalent levels of skin wettedness [9]. Therefore, an acclimatized individual can theoretically cover a greater proportion of their skin surface area in sweat, increasing the area over which evaporation can occur. This alone will lead to increases in evaporative capacity independent of other mechanisms.
Biophysical Factors that Affect Heat Stress
Just as environmental conditions can dictate the individual factors of heat gain or loss, physical characteristics of an individual can dictate relative responses to a given heat stress. Most commonly, the units of energy for the heat balance equation are expressed as W/m2 to normalize responses on the basis of body surface area (BSA) [11]. On the basis of the DuBois equation, this primarily allows for interindividual differences in stature (as an adjunct for BSA) to account for the amount of skin surface capable of interacting with the environment for heat exchange.
The other key demographic considerations in the determination of biophysical heat stress are body mass and body composition. Increased body mass not only increases the thermal inertia of an individual, it also increases the metabolic demand of a given exercise [20]. While theoretically the relationship between increases in body mass and body surface ratios is important, laboratory findings on the topic appear equivocal [21]. Similarly, body composition or increased body fat percentage can hypothetically influence the nature of heat exchange within the body. However, controlled laboratory work has found limited evidence for an independent effect of adiposity [22].
As nude exercise is not as popular as it used to be, the thermal properties of clothing and equipment must be considered alongside cutaneous factors. In addition to the microenvironments created between clothing and the body, clothing and equipment itself interact with the environment [23]. Fabrics and materials possess thermal and evaporative properties that interact in conjunction with human responses in proportion to the skin surface area covered [9]. Generally speaking, the greater surface area covered by a less “breathable” fabric, the greater heat stress [24].
Physiological Control of Heat Stress
Physiologic control of heat stress relies on the principles of heat balance discussed above and the integration of a neurologic autonomic control. This control, like many physiologic systems, can be divided into afferent and efferent responses, the former acting as a sensor and the latter acting as a regulator of the mechanisms the body employs. As in the cases of most diseases, exertional heat illnesses are often underpinned by either an inadequate or inappropriate physiological response.
Peripheral sensation of thermal stress is primarily believed to be mediated through the activation of temperature-specific transient receptor potential (TRP) channels [25]. These diffuse signaling pathways allow for region specific sensation of temperature and are primarily influenced by ambient temperature [26]. This afferent information is likely responsible for not only thermoeffector responses but also the perception of temperature and thermal comfort [27]. The abdomen also contains thermosensory neurons that proceed to the spinal column as a method of detecting deep body temperature [28].
The integration of human thermoregulatory information occurs in the hypothalamus, specifically the preoptic area [29]. Information from skin temperature sensors is integrated with temperature signals from the brain, spinal cord, and viscera alongside pyrogenic mediators (i.e., PGE2, which is activated as part of the immune response) [28]. It should be noted that this temperature information is obtained across the skin surface, viscera, and nervous system; therefore, no single anatomical point serves as a physiologic representation of internal body temperature.
In the context of hyperthermia , warm-activated neurons in the hypothalamus stimulate a GABAergic response which results in the activation of thermoeffector circuits and the body’s physiological mechanisms for heat dissipation [28]. The efferent responses of the thermoregulatory system during exercise in the heat are primarily eccrine sweating and cutaneous vasodilation. Both of these responses are sympathetically activated proportionally to afferent stimuli.
Sweating is the cholinergically activated result of sweat glands being activated via neural pathways descending from the sympathetic ganglia. This results in the activation of aquaporin channels that facilitate the entry of fluid into the sweat gland [30]. From there a hormone-mediated reabsorption of ions occurs as the fluid proceeds through the gland and onto the skin surface where it can be released for vaporization [31]. Since the vaporization of sweat liberates body fluid, prolonged or intense sweating has the ability to affect total body water and osmolality. Hypohydration has also been observed to diminish the sweating response at a given body temperature, demonstrating the compounding nature of hypohydration and heat stress [32].
During heat stress, the autonomic nervous system facilitates the delivery of blood to the skin’s surface to dissipate heat. As internal body temperature rises, relaxation of vasoconstrictor tone in non-glabrous (hair bearing) skin occurs to increase skin blood flow [33]. If convective heat loss via relaxation of vasoconstrictor tone cannot maintain heat balance, acetylcholine or other transmitters are released from sympathetic nerve endings to increase skin blood flow through active vasodilation [33]. The extent of this vasodilation, coupled with vasoactive effects of exercising muscle and the dehydrating effect of sweat, can limit the ability of the cardiovascular system to adequately perfuse tissues, resulting in cardiovascular drift [33, 34].
In glabrous (non-hair bearing) skin, withdrawal of sympathetic nervous activity increases blood flow through passive vasodilation [35]. Glabrous skin contains arteriovenous anastomoses (AVA), which directly connect small arteries and veins without capillaries in between. AVA can be found on the palms of hands, soles of feet, and lips. AVA primary function are to transfer heat from the skin’s surface to the environment since it does not contain capillaries for transportation of oxygen, nutrients, or waste products between blood and other tissues [35].
Acclimatization
Heat acclimatization (or acclimation in artificial environments) refers to the physiological adaptations that occur in response to repeated exposure to heat stress. Increasing exercise intensity in the heat exacerbates thermal strain (i.e., increased core temperature, heart rate, skin temperature), which can reduce exercise or work capacity. Heat acclimatization attenuates thermal strain through frequent heat exposures and produces positive adaptations that can preserve or improve performance [36]. HA induction over the course of 7–14 days can improve exercise performance in hot and temperate conditions through increased plasma volume, reduced core/skin temperature, improved thermal comfort, decreased heart rate, and improved sweating response [37]. The magnitude of changes that occur during and after HA is dependent on environmental conditions, exercise duration, exercise intensity, and HA induction method. Chapter 3, Predisposing Factors for Exertional Heat Illness, will discuss heat acclimatization in more detail.
Behavioral Thermoregulation

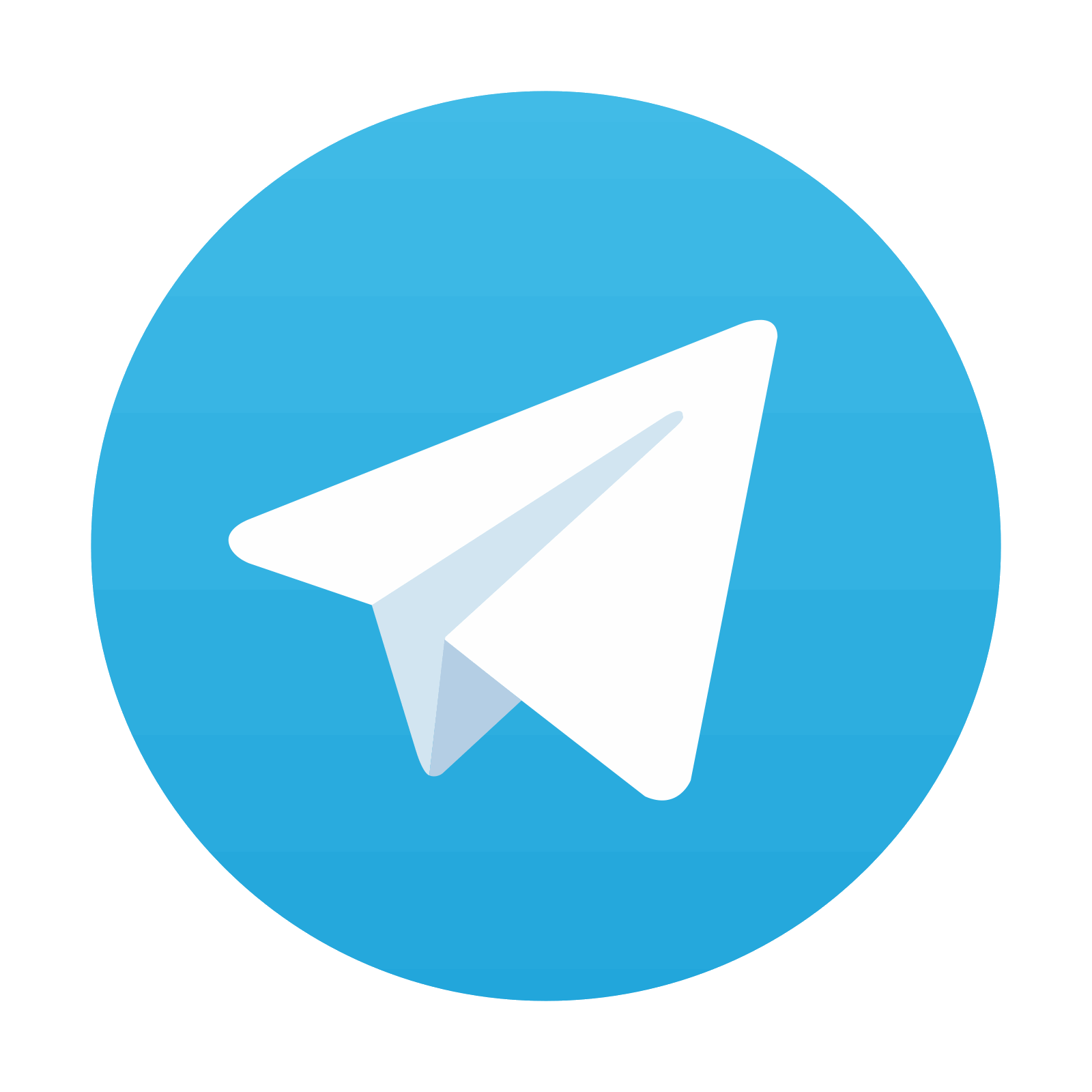
Stay updated, free articles. Join our Telegram channel
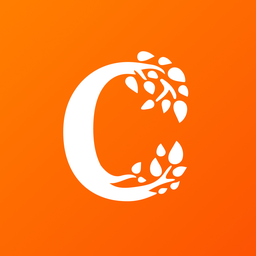
Full access? Get Clinical Tree
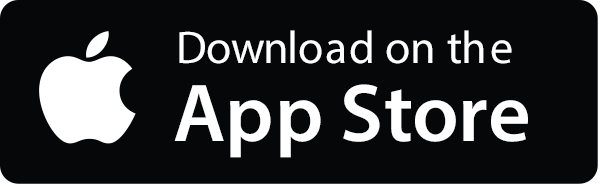
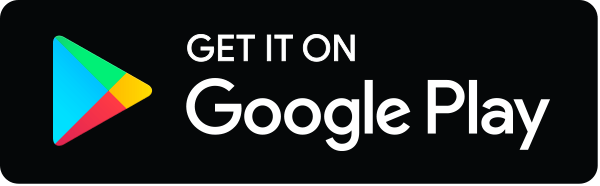