Regenerative Medicine: Implications in Rehabilitation
Martin K. Childers
Chad Markert
Anthony Atala
Jennifer Cann
George Christ
Mark Furth
Fabrisia Ambrosio
INTRODUCTION
An innovative interdisciplinary scientific field, termed regenerative medicine, focuses on new approaches to repairing and replacing cells, tissues, and organs and may involve the use of gene therapy (1, 2, 3, 4, 5, 6). Derived from the fields of biomedical engineering, developmental biology, nanotechnology, physiology, molecular and cellular biology, and surgery, regenerative medicine holds new promise for previously incurable conditions. Potentially, any disease or condition (acquired or genetic) that results in damaged, failing, or malfunctioning tissue may be amenable to regenerative medicine technologies. These technologies fall into two general categories (Fig. 82-1): (a) a focus on growing tissues and organs in vitro (i.e., tissue engineering) and subsequently implanting them into the patient; and (b) a focus to fully leverage the host’s regenerative in vivo capacity using cellular and/or gene therapies. This review concentrates on the latter approach in regenerative medicine.
In 2004, The Joint Commission on the Accreditation of Healthcare Organizations (JCAHO) warned that failure to address the severe shortage of organs could have ominous consequences for the American population. There is about one death every 30 seconds from organ failure, and complications and rejection of transplanted organs pose significant problems. The United Network for Organ Sharing (UNOS), which maintains a database on transplantation in the United States, reported that approximately 29,000 transplants were performed annually in 2006 and 2007. However, by mid-2008 the number of Americans on waiting lists for donated organs grew to nearly 100,000. These facts point to the importance of developing new therapeutic approaches for this huge unmet medical need. In this chapter, we describe just a few of the new approaches that the emerging field of regenerative medicine uses to address this need for organ and tissue replacement.
Cell Therapy: Regenerating Damaged Tissues In Vivo
Diseased tissue may be regenerated in vivo by transplantation of healthy cells that are able to replicate extensively. Progenitor and stem cells have this intrinsic ability, and are used in regenerative medicine to enhance or restore damaged tissue. According to data from the Centers for Disease Control, as many as 1 million Americans will die every year from disease that, in the future, may be treatable with tissues derived from stem cells (7). Diseases that might benefit from stem cell-based therapies include diabetes, heart disease, cerebrovascular disease, liver and renal failure, spinal cord injuries, and Parkinson’s disease. In the following section, we describe the classes of cells available for regenerative techniques and describe research advances using these cells in selected clinical conditions commonly encountered by rehabilitation clinicians.
Adult Stem Cells and Tissue Progenitor Cells
Adult stem cells are defined as any stem cell population that corresponds to a point in development subsequent to the inner cell mass (ICM), or possibly the slightly later epiblast, of embryos at the blastocyst stage, prior to gastrulation. In other words, adult stem cells are usually defined as stem cells found at any point in development beyond the pluripotent cells of early embryos. Adult stem cells are relatively abundant during fetal development and persist in tissues throughout adult life. Much of our current understanding of stem and progenitor cell biology derives from studies of a particular class of adult stem cells, namely those of the hematopoietic (blood forming) system. In fact, the therapeutic use of adult stem cells dates back to the first bone marrow transplant in 1956 (8). Early suggestions supporting the existence of cells that are capable of reconstituting the blood system came from experience with persons exposed to lethal doses of radiation during World War II. In the early 1960s, James Till and Ernest McCulloch in Toronto, Canada, found evidence for a specific subpopulation of cells in the bone marrow that could restore hematopoiesis after transplantation into irradiated mice. In the spleens of recipient animals, they observed large colonies and showed that these arose as clones from individual stem cells in the donor marrow that both could replicate to generate more stem cells and could give rise to multiple types of mature, differentiated blood cells (9,10). These two characteristics, termed self-renewal and multipotency, remain generally accepted as the defining features of stem cells. In the most complete demonstration of these properties, it has been shown that one hematopoietic stem cell has the capacity to completely repopulate both the lymphoid and myeloid blood cells of a lethally irradiated mouse and that single stem cells from such rescued animals can in turn reconstitute additional irradiated hosts over multiple sequential passages (11,12).
Human hematopoietic stem cells also have been characterized in detail and have similar properties to those in mice (13). They can be enriched using antibodies to surface markers such as CD34 and CD133 and can reconstitute the bone marrow and spleen of irradiated mice (severely immunodeficient mutant strains that do not reject human cells). In addition to bone marrow, umbilical cord blood has proven to be a valuable source of hematopoietic stem cells for therapeutic applications (14,15).
As in the bone marrow, the presence of stem cells in the intestinal mucosa was inferred initially from studies of recovery from radiation damage (16). Labeling experiments showed clearly that these stem cells are located near the base of crypts in the small and large intestines and give rise to the various mature cells of the gut. However, the intestinal stem cells have proven difficult to isolate and characterize outside of their in vivo niche. Recently, improved sorting and laser capture technologies have begun to facilitate the detailed phenotypic characterization of these cells (17,18).
Even under normal conditions, blood cells and intestinal epithelial cells turn over rapidly. The presence of stem cells in such adult tissues (the epidermal layer of skin serves as another example (19) has become widely accepted. The general model is that stem cells, found in special niches, serve as a self-renewing population responsible for the capacity for tissue restoration. The stem cells give rise to progenitor cells, which can still multiply many times but are unable to indefinitely self-renew or to completely reconstitute a tissue. The progenitors, in turn, are precursors of terminally differentiated cells that generally no longer divide (20,21). Operationally, it can often prove difficult to distinguish rigorously between stem and progenitor cells, as adequate long-term in vivo reconstitution assays are not always available. Both classes are likely to have therapeutic value.
Stem and progenitor cells also have been identified in tissues and organs in which cells normally turn over much more slowly than in the bone marrow, gut, or skin. These include skeletal muscle, liver, kidney, endothelial cells lining blood vessels, and many more (22,23). Remarkably, even specialized cell types that for many years were believed incapable of any degree of restoration in adults, such as neurons of the central nervous system (CNS) and cardiac myocytes, now appear to have corresponding stem cells that persist throughout adult life (24,25).
Mesenchymal Stem Cells
While many adult stem cells appear specific to the tissue or organ from which they are obtained, others are more broadly distributed and also may have less restricted differentiation potential. An example with great clinical potential is the mesenchymal stem cell (MSC), a multipotent cell that can give rise to bone, cartilage, adipocytes (fat cells), and fibroblasts. MSCs were first identified as a stromal population in the bone marrow, distinct from hematopoietic stem cells (8,10,13,14). However, similar cells have been found throughout the body, and there are now clear indications that MSCs are perivascular cells associated with blood vessels (26). An accessible, abundant source of such cells is fat tissue obtained by liposuction (27) and also from placental tissue (unpublished observations). Although termed “stem cells,” the MSCs do not display indefinite self-renewal capacity and cannot be routinely expanded in culture beyond approximately seven or eight passages. A single bone marrow donation can yield thousands of potential clinical doses of MSCs but not an unlimited supply. A subset of MSCs from bone marrow appears to have greater capacity to proliferate, perhaps “indefinitely,” and also to differentiate beyond the mesenchymal cell lineages. Verfaillie and colleagues have termed them multipotent adult progenitor cells (MAPC). The MAPCs been reported to differentiate in vitro into numerous tissue types and also to contribute to a wide array of adult tissues and cell types after injection into a developing embryo at the blastocyst stage (14).
Some adult stem and progenitor cell populations have proven difficult to propagate in culture. For example, despite the great effort that has been expended studying hematopoietic stem cells, there has been little success in expanding their numbers in the laboratory. Other stem cell populations, such as satellite cells of skeletal muscle, multiply substantially in culture but then show diminished regenerative capacity when transplanted in vivo (28). Nonetheless, some cell populations that must include adult stem and progenitor cells have been expanded sufficiently for certain clinical applications. Examples include cells from human foreskin utilized for living skin products (29) and urothelial and smooth muscle cells used in tissue-engineered constructs for bladder augmentation (30). Furthermore, certain purified stem cell populations have been grown continuously in culture for many months—at least from a practical perspective, this may be viewed as “unlimited” self-renewal. Keys for expanding a number of adult stem cells have been enrichment of rare cells using selection with antibodies to surface markers, and the use of serum-free defined medium, carefully chosen growth factors, and, in some cases, extracellular matrix components for their expansion. For example, neural stem cells can be isolated from brain tissue by positive selection for CD133 and negative selection for markers found on contaminating cell types (31). When cultured in
defined medium with fibroblast and epidermal growth factors, they grow as “neurospheres” that maintain a significant proportion of multipotent stem cells able to give rise to neurons, astrocytes, and oligodendrocytes (32,33). Recently, conditions were reported for the isolation of human hepatic stem cells, by immunoselection for the epithelial cell adhesion molecule (EpCAM), and their expansion in culture for over 150 population doublings (34, 35, 36). These cells are precursors to hepatocytes and bile duct epithelium and may have broader potential to generate other endodermal cell types. Spermatogonial stem cells provide yet another example of a growing list of adult stem cells (albeit for a germ cell fate) that can be propagated extensively in a defined medium (37).
defined medium with fibroblast and epidermal growth factors, they grow as “neurospheres” that maintain a significant proportion of multipotent stem cells able to give rise to neurons, astrocytes, and oligodendrocytes (32,33). Recently, conditions were reported for the isolation of human hepatic stem cells, by immunoselection for the epithelial cell adhesion molecule (EpCAM), and their expansion in culture for over 150 population doublings (34, 35, 36). These cells are precursors to hepatocytes and bile duct epithelium and may have broader potential to generate other endodermal cell types. Spermatogonial stem cells provide yet another example of a growing list of adult stem cells (albeit for a germ cell fate) that can be propagated extensively in a defined medium (37).
Embryonic Stem Cells
In contrast to adult stem cells, embryonic stem (ES) cells show essentially unlimited capacity for self-renewal in culture and should be able to give rise to any of the more than 200 cell types in the body (with the exception of certain extraembryonic cell types). The isolation of ES cells from the inner cell of blastocyst stage mouse embryos was reported in 1981 by Martin (38) and by Evans and Kaufman (39), based on many years of prior work on transformed embryonal carcinoma cells from teratocarcinoma tumors. Successful isolation of ES cells from human blastocysts was not achieved until 17 years later, by Thomson et al. (40).
ES cells have great therapeutic potential, but there are also significant barriers that must be overcome for their implementation in the clinic. ES cells are pluripotent, that is, they have the ability to form tissue representing all three embryonic germ layers—the ectoderm, mesoderm, and endoderm. Thus, the ES cell has the intrinsic ability to give rise to daughter cells that can form virtually any tissue in the body. This was demonstrated convincingly by the finding that mouse ES cells injected into developing embryos can contribute to all adult cell types, including germ cells (40,41). Human ES cells have been induced to generate representatives of all three germ layers in culture and are widely assumed to have the same degree of pluripotency as mouse ES cells.
The great plasticity of ES cells can also represent a drawback to their use, because it may prove more difficult to induce them to yield a single desired cell type exclusively than when starting with lineage-committed adult stem cells. However, significant progress has been made in the directed production of many specialized cells, or at least lineage-specific progenitors, from mouse and human ES cells (42, 43, 44). Examples include cells of neuronal, epidermal, cardiac myocytic, hematopoietic, endothelial, hepatic, endocrine pancreatic, and germ cell lineages. Another important problem that must be overcome is that undifferentiated ES cells form teratoma tumors (38, 39, 40,44). Therefore, for clinical use it will be essential to differentiate the cells quantitatively before implantation and to rigorously exclude the presence of residual stem cells. Finally, progress is being made in the derivation of human ES cells in the absence of xenogeneic feeder cells and animal proteins that may pose risks for clinical application.
The derivation and certain potential uses of human ES cell lines have engendered a significant ethical debate, especially because embryos, though donated for research, are destroyed in order to isolate the ICM (45,46). Technological advances may offer at least a partial resolution to the ethical controversy. For example, human ES lines can now be developed from single-cell biopsy at the eight-cell stage of development, without compromising the viability of the embryo, similar to a widely used procedure for prenatal genetic diagnosis (47). The development of ES-like cell lines by reprogramming of normal adult cells ultimately may put the debate to rest by providing pluripotent cells entirely without the use of donated embryos or oocytes (see below).
Cells Derived From Somatic Cell Nuclear Transfer
Somatic cell nuclear transfer (SCNT) involves the removal of an oocyte nucleus in culture, followed by its replacement with a nucleus derived from a somatic cell obtained from a patient. Activation with chemicals or electricity stimulates cell division up to the blastocyst stage, at which time the ICM is isolated and cultured, resulting in ES cells genetically identical to the patient. In animal studies, nuclear transferred ES cells derived from mouse fibroblasts, lymphocytes, and olfactory neurons are pluripotent and can generate live pups, showing the same developmental potential as fertilized blastocysts (48, 49, 50). The resulting ES cells are perfectly matched to the patient’s immune system.
Although promising, SCNT has certain limitations that require further improvement before clinical application. Animal studies have shown that blastocysts generated from SCNT and implanted into a uterus can produce an infant clone of the donor. In 1997, for example, a sheep named Dolly was derived from an adult somatic cell using SCNT (51). This form of reproductive cloning is banned in most countries for human applications. In contrast, therapeutic cloning can generate ES cell lines whose genetic material is identical to that of their source. In therapeutic cloning, blastocysts are grown until a 100 cell-stage where ES cells can be obtained, but the blastocysts are never implanted into a uterus. However, this technique has not been shown to work in humans. Initial failures and fraudulent reports of nuclear transfer in humans reduced enthusiasm for human applications (52, 53, 54). However, it was recently reported that nonhuman primate ES cell lines were generated by SCNT of nuclei from adult skin fibroblasts (55,56).
Cellular Reprogramming
Reprogramming is a technique that involves dedifferentiation of adult somatic cells to produce pluripotent stem cells without the use of embryos. Cells generated by reprogramming are theoretically identical to somatic cells that would not be rejected by the donor. This method also avoids nuclear transfer into oocytes. Takahashi and Yamanaka (57) were the first to report that both embryonic and adult mouse fibroblasts can be reprogrammed into an induced pluripotent state (IPS).
They identified four key genes required to bestow ES cell-like properties in fibroblasts: OCT3/4, SOX2, c-MYC, and KLF4. Reprogrammed cells were selected via drug resistance. The resultant IPS cells possessed the immortal growth characteristics of self-renewing ES cells, expressed genes specific for ES cells, and generated embryoid bodies in vitro and teratomas in vivo. When the IPS cells were injected into mouse blastocysts, they contributed to a variety of diverse cell types, demonstrating their developmental potential. It was recently reported that reprogramming by transduction of four defined factors can be done with human cells (58). Retrovirus-mediated transfection of OCT3/4, SOX2, KLF4, and c-MYC generates human IPS cells that are similar to human embryonic stem (hES) cells in terms of morphology, proliferation, gene expression, surface markers, and teratoma formation. Others (58) have shown that retroviral transduction of OCT4, SOX2, NANOG, and LIN28 could generate pluripotent stem cells without introducing any oncogenes (c-MYC) (59). An alternative approach would be to use a transient expression method, such as adenovirus-mediated system (59,60). Modified donor cells may not be necessary to generate IPS cells (60).
They identified four key genes required to bestow ES cell-like properties in fibroblasts: OCT3/4, SOX2, c-MYC, and KLF4. Reprogrammed cells were selected via drug resistance. The resultant IPS cells possessed the immortal growth characteristics of self-renewing ES cells, expressed genes specific for ES cells, and generated embryoid bodies in vitro and teratomas in vivo. When the IPS cells were injected into mouse blastocysts, they contributed to a variety of diverse cell types, demonstrating their developmental potential. It was recently reported that reprogramming by transduction of four defined factors can be done with human cells (58). Retrovirus-mediated transfection of OCT3/4, SOX2, KLF4, and c-MYC generates human IPS cells that are similar to human embryonic stem (hES) cells in terms of morphology, proliferation, gene expression, surface markers, and teratoma formation. Others (58) have shown that retroviral transduction of OCT4, SOX2, NANOG, and LIN28 could generate pluripotent stem cells without introducing any oncogenes (c-MYC) (59). An alternative approach would be to use a transient expression method, such as adenovirus-mediated system (59,60). Modified donor cells may not be necessary to generate IPS cells (60).
Stem Cells Derived From the Amniotic Fluid and Placenta
The amniotic fluid is known to contain a heterogeneous population of cell types derived from the developing fetus (61,62). Cells from this population have been shown to differentiate into adipocytes, osteocytes, neurogenic, and endothelial cells (63, 64, 65, 66). Importantly, a subpopulation of OCT4-positive cells have been detected in the amniotic fluid (64,67). Recently, the isolation of human and mouse amniotic fluid-derived stem (AFS) cells was reported. These cells are capable of extensive self-renewal and give rise to adipogenic, osteogenic, myogenic, endothelial, neurogenic, and hepatogenic lineages (68). In this respect, they meet a commonly accepted criterion for pluripotent stem cells.
AFS cells represent approximately 1% of the cells found in the amniotic fluid. The same cells can also be found in the placenta. These cells are immunoselected for cells that express the surface antigen c-kit (CD117), the receptor for stem cell factor (69). AFS cells express embryonic markers such as OCT4 and SSEA4 but not other markers of ES cells. They also express mesenchymal and/or neuronal markers such as CD29, CD44, CD73, CD90, and CD105. Clonal analyses using retrovirally marked human lines confirmed that differentiated cells of various types can be derived from a single AFS cell (68).
In addition to demonstrating that differentiated cells express lineage-specific markers, we have shown that AFS cells give rise to specialized functions (68). Cells differentiated down a neuronal pathway secreted glutamine or expressed G-protein-gated inwardly rectifying potassium channels. Cells of the hepatic lineage secreted urea and a-fetoprotein, while osteogenic cells produced mineralized calcium.
The recent discovery of a stem cell population in the amniotic fluid and placenta offers a promising alternative source of stem cells for cellular therapy. The full range of adult somatic cells that AFS cells can produce remains to be determined, but their ability to differentiate into cells of all three embryonic germ layers and their high proliferation rate are two advantages over most adult stem cell sources. AFS cells represent a new class of stem cells with properties somewhere between embryonic and adult stem cell types. However, unlike ES cells, AFS cells do not form teratomas and are easily obtained without destruction of embryos. AFS could be use for both autologous and allogeneic.
Rehabilitation Patients and Potential Impact of Stem Cell Therapy
Spinal Cord Injury
A target for cellular therapy in spinal cord injury (SCI) arises from the fact that damaged neurons of the CNS are unable to self-repair after damage. Transplantation of MSCs into damaged neurons of the CNS may create an anti-inflammatory microenvironment permissive to outgrowth of the proximal axon stump (70, 71, 72). Intriguing studies on olfactory ensheathing cells, specialized glial cells that surround sensory axons in the nose, suggest that when these cells are used to reestablish previously damaged pathways, severed axons have a structural connection onto which they may regenerate (73). According to the pathway hypothesis, olfactory ensheathing cells provide a “bridge” along which nerve fibers, with limited latent growth potential, may regrow. Although functional improvement after SCI has been reported in animal models following cell transplantation (74,75), only a few clinical studies of cell therapy approaches to SCI are reported. For example, a trial of bone marrow cell transplantation into SCI patients (n = 35) reported no safety concerns, yet the data indicated only meager functional improvements (76). Of interest, two SCI patients who received both autologous MSC transplantation and rehabilitation therapy demonstrated significant functional improvement (77). The question of how rehabilitation treatment might benefit SCI patients receiving stem cell transplantation will be an important area for future investigation.
Brain Injury
Improvement in neurological function was reported following MSC transplantation in brain injured rats. In one study, 2 × 106 human MSCs were infused intravenously (78) without evidence of tissue rejection. In rat models of traumatic brain injury (79) and cerebral ischemic stroke (80,81), cell chemotaxis, or homing, was observed. Homing refers to the directional migration of cells, regulated by chemokines associated with tissue damage. The ability of stem cells to migrate to diseased/injured cells, tissues, and organs is central to therapeutic utility of MSC transplantation. MSCs are naturally home to areas of tissue damage, and thus offer an attractive source of stem cells for in vivo regenerative applications. The mechanisms governing the efficiency of MSC homing are the topic of a number of studies (82, 83, 84).
Myocardial Infarction
Animal models of myocardial infarction (MI) have demonstrated migration of MSCs to the injured heart with subsequent improvement in cardiac function (85, 86, 87, 88, 89, 90, 91
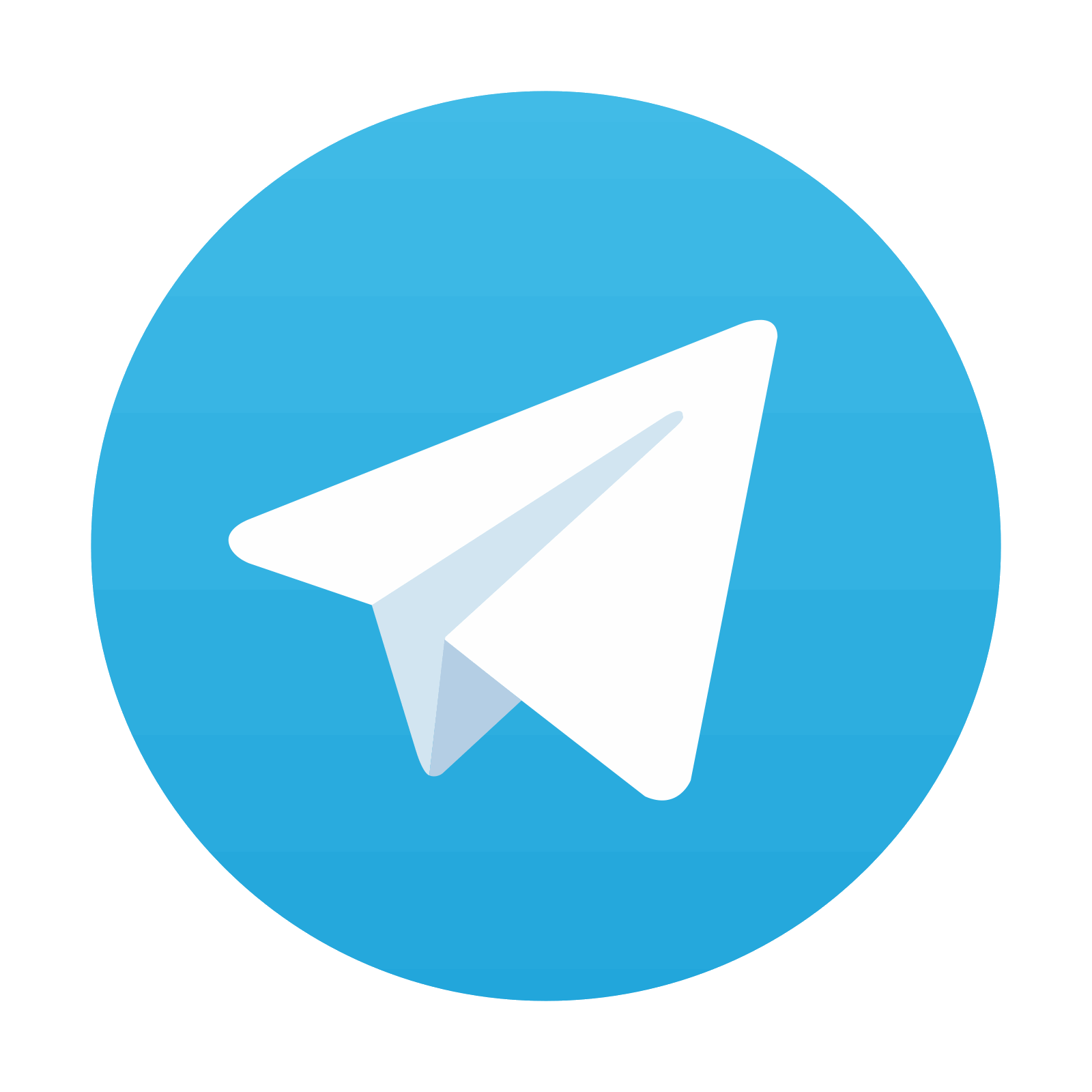
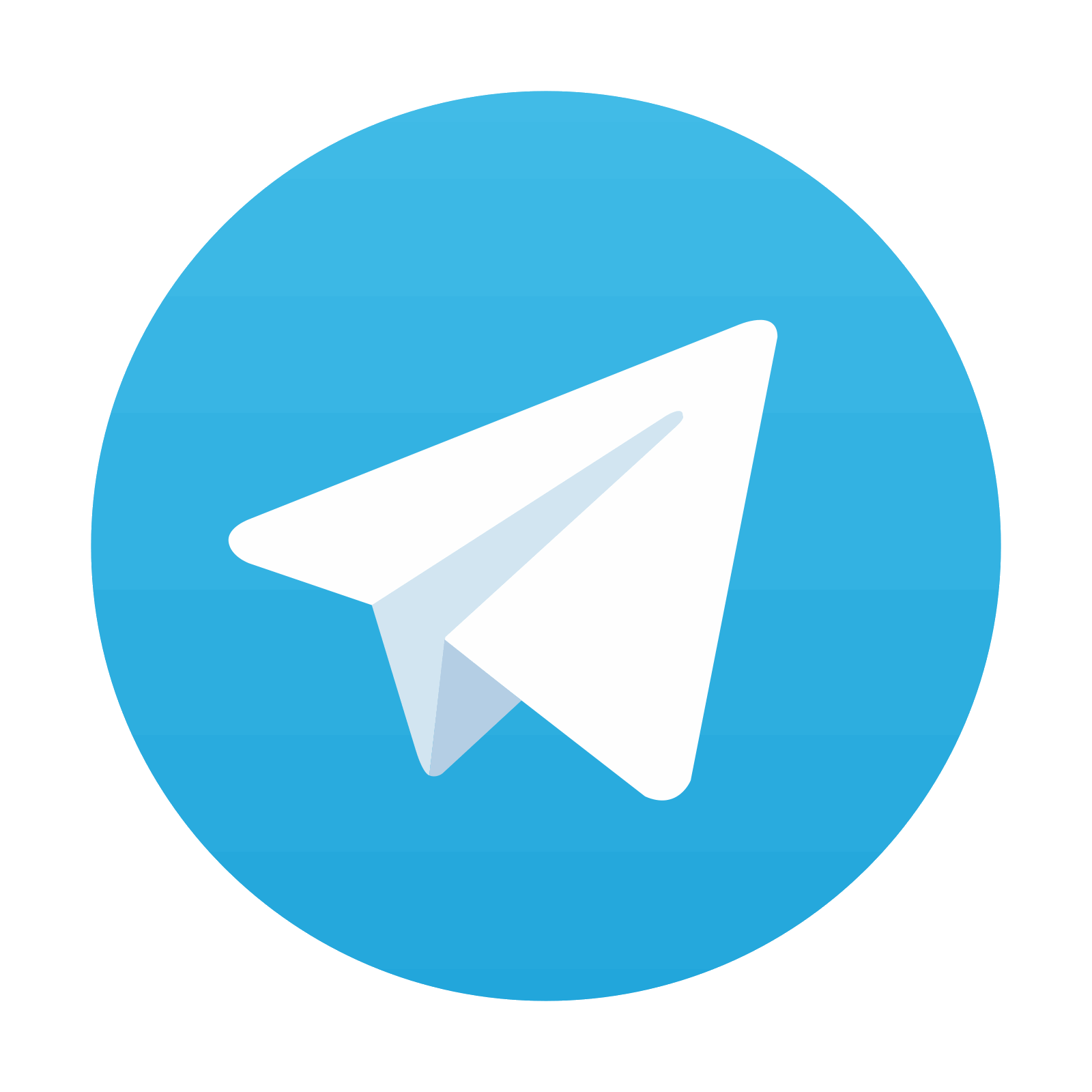
Stay updated, free articles. Join our Telegram channel
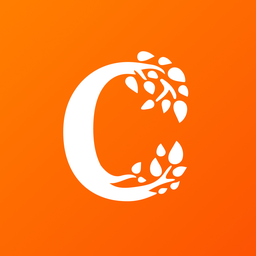
Full access? Get Clinical Tree
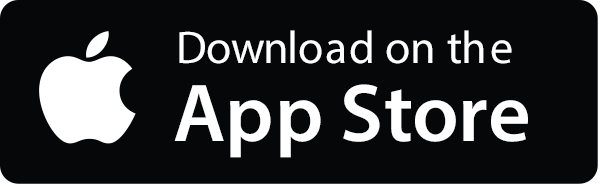
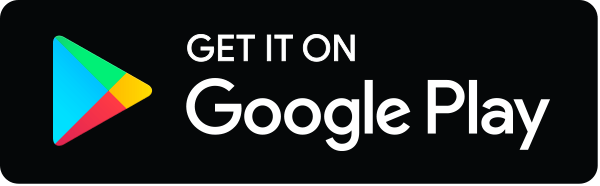
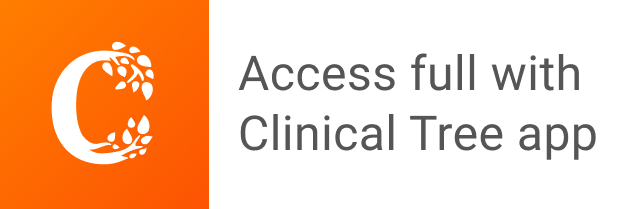