4 Primary malignant bone tumors of the spine present significant treatment challenges with regard to achieving local tumor control while preserving neurologic function. The most common primary tumors involving the spinal column are chordomas, chondrosarcomas, and osteogenic sarcomas. Significant advances in both surgery and radiation are redefining their roles in the treatment of these lesions. Advanced surgical techniques for en-bloc resection of primary spine tumors improves the ability to achieve marginal or wide curative resections1–3; however, many tumors are not amenable to en-bloc resection. Conventionally fractionated radiotherapy is frequently used as an adjuvant to surgery. In most cases, conventional external beam radiation is not an effective primary therapy for many primary spine tumors. The poor long-term outcomes after conventional radiation therapy is likely related to the relatively low dose of radiation that can be given near the spinal cord. Concern about spinal cord tolerance has typically limited radiation doses to less than 54 Gy in standard fractions.4,5 Low-dose areas within the irradiated field are the most likely to result in disease recurrence.6 Three radiation techniques are currently being used to increase the dose to the tumor while sparing normal tissue tolerance in an attempt to treat primary spine tumors: particle beam treatment, such as proton beam therapy; brachytherapy; and high-dose conformal photon therapy, such as image-guided intensity-modulated radiation therapy (IMRT) or stereotactic radiosurgery (SRS). Although class 1 evidence is lacking, mounting research demonstrates the contribution of high-dose radiation to achieve local tumor control for various spine tumor pathologies. Recently, the ability to deliver SRS using image-guided IMRT has proven useful in the neoadjuvant and postoperative setting. The role of en-bloc resection to achieve a marginal or wide resection is well established for extremity sarcomas.7–9 Over the past 10 years, spine surgeons have developed techniques for en-bloc resection of primary spine tumors that improves cure rates compared with piecemeal, curettage techniques. These techniques are safe for patients with isolated vertebral body or posterior element disease. Boriani et al10 published a surgical series regarding en-bloc spondylectomy for low-grade chondrosarcoma of the mobile spine in 22 patients. Of the 12 patients who underwent en-bloc excision, 9 (75%) maintained local control at a median follow-up of 81 months (range, 2 to 236 months). Of the three recurrences, two had contaminated margins at surgery from epidural disease. The remaining 10 patients underwent curettage resection and by definition had intralesional resections with positive histological margins. At a median follow-up of 36 months, the recurrence rate was 100%, and 80% died of disease.10 Of note, in this group, three patients received conventional-dose external beam radiation and no patients received high-dose conformal radiation with proton beams or image-guided IMRT. Although curative resections are feasible in some patients, many patients present with factors that may preclude en-bloc resection that achieves negative histological margins. En-bloc resection of the isolated vertebral body or posterior elements is technically feasible. However, according to the Weinstein–Boriani–Biagini (WBB) classification,11 patients who present with epidural tumor, multilevel large paraspinal masses, or circumferential bone disease are not candidates for marginal or wide excisions (i.e., en bloc with negative margins). The feasibility of achieving a wide or marginal excision is limited by the risk of neurologic or adjacent structure injury. For example, resecting the dura en bloc with a specimen could possibly provide a margin on epidural tumor. Unfortunately, the loss of spinal fluid buffering the spinal cord increases the probability of injury to the spinal cord and complications of cerebrospinal fluid (CSF) leak. If tumor is spilled during resection, there seems to be a higher probability of intradural seeding as well.12 In a series of 59 spine sarcomas reviewed using the radiographic criteria established by the WBB classification, approximately 15% of patients were candidates for en-bloc excisions that could achieve a wide or marginal margins. As noted in extremity sarcomas, once the tumor is violated, the risk of recurrence significantly increases.7 However, the treatment of sarcomas at other sites has demonstrated the utility of adjuvant high-dose radiation in the setting of microscopic or gross residual disease post resection.13 Radiation therapy is an extremely important modality in the treatment of primary spine tumors. However, the relative radioresistance of these tumors requires doses well above spinal cord tolerance for durable local tumor control. From the paradigm of extremity sarcomas, radiation doses of greater than 60 Gy in 2-Gy fractions are required for control of positive microscopic margins and greater than 70 Gy for gross residual disease.14 Traditional concepts of spinal cord tolerance using conventional radiation techniques establishes the TD5/5 (the tolerance dose at which there is a 5% probability of a complication within 5 years) at 50 Gy in 1.2- to 2-Gy fractions above which there appears to be a significantly increased risk of developing radiation myelitis.15 Toxicity to the spinal cord may also be associated with the length of cord irradiated. In addition to the spinal cord, toxicities to paraspinal structures, such as the bowel, the kidneys, and the esophagus, need to be considered. Unfortunately, the radiation tolerances of these organs range from 23 to 60 Gy. Thus, technologies and techniques that minimize the radiation dose to critical organs and allow high dose to the tumor are essential for meaningful radiation treatment of spine malignancies. Advances in radiation technology, including the introduction of hadrons (high-dose protons or charged particles, including carbon ions, helium, or neon), have led to higher doses of radiation being delivered to the target volume with reduced tissue injury and improved radiobiological effect.16 There are many important similarities as well as differences between IMRT and proton beam radiotherapy. The rationale for using proton beams is the excellent dose distribution at the tumor target and virtually no exit dose beyond the target volume. The Bragg peak phenomenon characteristic of proton beam radiation results in an extremely steep dose falloff that can be measured over a course of millimeters (Fig. 4.1). The biological impact of proton beam treatment and IMRT is relatively minor. The conversion factor commonly used to compare the effectiveness of proton beam treatment with photon radiotherapy is 1.1. Thus, 1 Gy of proton radiation is biologically equivalent to 1.1 Gy of radiation from a cobalt source, or 1.1 cobalt gray equivalents (CGE). The advantage of proton beam treatment does not lie in an enhanced effect of protons against tumor cells. Rather, the advantage lies primarily in the lack of exit dose after the Bragg peak (Fig. 4.2). If the Bragg peak occurs just before the spinal cord, then the spinal cord receives minimal radiation dose, in stark contrast to photon radiation, for which exit dose is significant. If manipulated properly, proton beams can deliver higher doses to a tumor in close proximity to the spinal cord while exposing the spinal cord to minimal radiation doses. IMRT always delivers higher radiation dose to spinal cord adjacent to a spinal tumor, because there is no Bragg peak effect with photons, and some photons will pass through the tumor into the spinal cord. IMRT can deliver radiation dose to the tumor up to what is considered the cord tolerance. Fig. 4.1 The Bragg peak effect for 250-MeV protons. Note that there is no dose at a depth beyond the Bragg peak. In most cases, doses of up to 74 Gy can be given to a vertebral body tumor while limiting the spinal cord to 50 to 54 Gy. Thus, IMRT is able to deliver similarly high doses of radiation to the tumor, but the spinal cord doses are potentially lower with the use of proton beams.17 Proton beam radiation has a distinct disadvantage in patients with implanted spinal fixation hardware. The greater density of metallic implants can affect the range of protons up to 10 mm and the dose greater than 10% in the high-dose region.18 When the spinal cord may only be a few millimeters away from the high-dose region, the uncertainties associated with titanium implants are unacceptable. Monte Carlo simulation techniques are necessary to obtain accurate dosimetry in the setting of spinal instrumentation. On the other hand, photon radiation and IMRT dosimetry are minimally impacted by surgical hardware. Heavy ions such as carbon ions provide biological, in addition to physical, advantages compared with photons or protons, in terms of their high relative biological effectiveness and reduced oxygen-enhancement ratio in the tumor region.19 Hypofractionated carbon ion therapy is a promising treatment approach for large sacral chordomas.20 There has been considerable experience with the treatment of neuraxis tumors using proton beams to deliver fractionated therapy. Proton beam radiotherapy’s main limitation is the high cost of building and maintaining such facilities. The cost/benefit ratio of proton beam treatment is extremely controversial, but the cost of proton therapy is expected to decrease rapidly. Currently it is approximately twice as expensive as IMRT.21 Excellent results for uveal melanoma have been reported using proton beam therapy in a hypofractionated manner (median dose 70 CGE in five fractions),22 but data reporting the use of single-fraction radiation with proton beam therapy for the management of tumors of the neuraxis is lacking. Fig. 4.2 Axial proton beam dose distribution for a sacral lesion entering posteriorly. Note the lack of exit dose into the pelvis. Proton-beam therapy combined with wide en-bloc excision is the accepted treatment standard in the management of chordomas at a number of cancer centers, especially in patients with a primary tumor as opposed to disease recurrence.16 Hug23 reported radiation results from 47 patients with primary or recurrent osteogenic and chondrogenic tumors treated with combined proton/photon therapy. The 5-year local control rates were 53% for chordoma, 50% for osteogenic sarcoma, and 100% for chondrosarcoma.23 Of the six failures, five were in field recurrences and one out of field. A trend was noted toward improved local tumor control in patients receiving greater than 77 CGE. The failures were seen primarily in patients with less than 77 CGE delivered to the tumor volume. Austin et al6 reported a case series of 141 patients treated for chordoma and chondrosarcoma of the skull base and cervical spine, using mixed proton/photon beam therapy at a median of 69 CGE. At a median follow-up of 3 years, 26 failures were noted. Of these failures, 23% received the prescribed tumoral dose. However, 77% of failures occurred in areas that received less than the prescribed tumoral dose, the majority of which were in regions constrained by normal tissue tolerance. All tumors that failed in the high-dose region had volumes greater than 75 cc. Patients with cervical spine disease had a higher rate of recurrence (10 of 26) and larger tumors (average volume of 102 cc) than those with base of skull disease (16 of 115) with an average volume of 63 cc. These studies underscores the challenges of delivering uniformity of prescribed dose and the treatment of large-volume tumors in close proximity to dose-limiting neural structures.6 Total doses in excess of 70 Gy can be routinely administered to paraspinal tumors using photon IMRT techniques while maintaining spinal cord doses at the same level achieved with proton beams (Fig. 4.3). Typically, five to seven radiation beams are utilized around a single isocenter. An inverse treatment planning algorithm is used to assign target doses to target volumes, and penalties are given to excessive doses to avoidance organs at risk. Multiple iterations of the number of beams, beam angles, and beam energies are utilized to arrive at a best possible solution that meets the dose goals for the target volumes and dose-sensitive nearby organs. Each individual beam intensity is modulated during treatment in a way that the sum total of all the beams results in a dose cloud that conforms to the three-dimensional characteristics of the target volume and typically requires very high dose gradients near the spinal canal and esophagus, in the case of cervical and thoracic lesions. Kidneys and bowel loops are common avoidance structures in the lumbar spine. Fig. 4.3 Dose distribution for a T11 osteosarcoma that is circumferential around the spinal canal. The prescribed dose was 72 Gy and the maximum dose to the spinal cord was 54 Gy. At Memorial Sloan-Kettering Cancer Center (MSKCC), we have reported the use of high-dose IMRT for the management of primary malignancies of the mobile spine. The actuarial local control was found to be 75%, with follow-up extending to a maximum of 40 months. No patient experienced myelitis. The median dose was 6,600 cGy (range, 5,400–7,200 cGy).24 We have treated seven chordomas and five chondrosarcomas, two of which were highgrade and the remaining intermediate or low grade. At a median follow-up of 18 months, the local control rate for chordomas was 86% and for chondrosarcomas was 80%, with the single failure occurring in a high-grade patient. The follow-up is too short to draw meaningful conclusions, but fractionated therapy using image-guided IMRT is possible at doses similar to those reported for proton beam therapy.25 Brachytherapy has also been used to treat primary tumors. Iodine 125 has commonly been used to treat positive microscopic disease or minimal residual gross disease following tumor resection. Good results have been reported in the treatment of paraspinal tumors and epidural disease.26,27 Rogers et al27 reported a series of 25 patients who were implanted intraoperatively with 125I seeds. Twenty-two (88%) failed prior external beam radiation therapy. At a mean follow-up of 19.2 months, four patients demonstrated local failure and the 3-year actuarial control rate was 72.9%. No radiation toxicity was seen in this study. However, radiation myelitis has been reported 34 months following the routine administration of 131I for metastatic thyroid cancer. The level of myelitis entailed significant epidural disease that was nearly circumferential, likely resulting in a high surface dose to the spinal cord over a short period of time.28 DeLaney et al29 have developed applicators for the delivery of high-dose radiation using iridium 192 and yttrium 90.29 90Y is a pure B-emitter and ideal for delivering high-dose radiation (e.g., 7.5 to 15 Gy) to the dura without toxicity to the spinal cord. The 90Y dose penetrance is 29% at 2 mm and 9% at 4 mm. This provides an adequate margin on the spinal cord, if the gross tumor has been resected in the absence of a spinal fluid leak. In this series, seven of eight patients were treated for sarcoma, six of whom were controlled at a median of 24 months. No radiation myelitis has been seen to date. 90Y may ultimately prove to be excellent as an adjuvant therapy for radioresistant tumors with epidural disease when combined with image-guided IMRT or proton beam therapy. Image-guided IMRT uses dose painting to lessen the dose at the spinal cord margin in order to spare spinal cord tolerance, potentially underdosing this area. 90Y may improve the dose distribution for epidural disease and facilitate treatment planning and delivery of image-guided IMRT. The limitation of 90Y is that the applicators are custom made for each patient and the isotope has a very short half-life. If the epidural tumor resection is more extensive than predicted by magnetic resonance imaging (MRI) or the case is delayed several days, the plaque may be wasted. Memorial Sloan-Kettering Cancer Center is pioneering the use of a similar dural plaque application of intraoperative brachytherapy for the treatment of metastatic and primary spine tumors. In this type of brachytherapy a thin piece of silicone is coated with radioactive phosphorus (32P). 32P has a two week half life and is slightly less penetrating than 90YT, both important advantages for 32P. The plaque is temporarily inserted directly onto dura contaminated with tumor cells during surgery and removed before the operation is over (Fig. 4.4). Similar to the 192Ir and 90Y used by Delaney et al,29 this allows delivery of high doses to the cord surface (25 Gy) while sparing the nearby spinal cord to doses typically less than 2 Gy. High-dose-rate (HDR) brachytherapy can also be delivered to spine tumors using small catheters while the patient is under anesthesia. As with other forms of brachytherapy, this application may assist in the dosimetric planning challenges of radiotherapy.30 32P has been used successfully for years as a local treatment for other types of tumors, but its safety and utility in the management of primary spinal axis tumors is still under investigation at MSKCC.31 Stereotactic spine radiosurgery delivers an entire course of radiation in one to five fractions. Hence, the dose of radiation per fraction is much higher than with conventional radiotherapy, and extreme accuracy and precision is crucial. IMRT techniques are typically used to generate the necessary conformity to limit doses within safe levels to nearby organs at risk. Image-guided technology, such as on-board cone beam computed tomography (CT) scanners, which are integrated into the radiation delivery platform, provide three-dimensional imaging and spatial data to ensure that the radiation is given exactly to the right place in near real time. The role for stereotactic radiosurgery in the treatment of primary tumors is yet to be determined, and experience is limited compared to proton beam therapy. Theoretically, high-dose single-fraction therapy may improve local tumor control compared to standard fraction therapy. From a biological standpoint, tumor histologies with low α/β ratios, such as sarcomas, respond better to larger fraction sizes. Preclinical evidence suggests that single-fraction therapy greater than 8 to 10 Gy results in apoptosis of tumor cells based on the acid sphingomyelinase pathway.30 Microvascular damage in the tumor from high-dose fractions likely contributes to cell death. The radiobiological benefit may be most pronounced in tumor types traditionally considered resistant to standard fractionated radiation therapy. A construct called the biological effective dose (BED) (Table 4.1) has become a widely accepted way to compare the potency of different radiation dose schedules.32 Although the assumptions made for BED calculations based on linear quadratic formalism are not likely to be accurate at high doses per fraction, such as in radiosurgery,33 a comparison of different dose schedules demonstrates the significant advantage of increasing the dose of radiation per fraction for radioresistant tumors (α/β = 2) compared with relatively sensitive tumors (α/β = 10). Thus increasing the fraction size is a form of biological dose escalation. Based on this simplified analysis, a course of proton beam radiotherapy giving 76 CGy in 2-Gy fractions would give the BED of 152 Gy for radioresistant tumors. In order for such high biological doses of radiation to be administered safely, it is critical that radiation be delivered in a very conformal manner with high accuracy and precision, to prevent the exposure of dose-sensitive normal tissues to high doses. Image-guided techniques34 coupled with IMRT have provided the avenue for such treatment. The extremely high local control rates and very low toxicity associated with metastatic renal cell carcinoma spine lesions treated with high-dose single-fraction radiosurgery appears to support this paradigm.35 Stereotactic radiosurgery is currently being used as neoadjuvant therapy at MSKCC. For tumors such as chordoma, chondrosarcoma, and liposarcoma, neoadjuvant radiation may decrease the possibility of tumor dissemination from intralesional resection. This may also benefit patients who ultimately undergo en-bloc resection with negative histological resections in which tumor dissemination is sometimes found. Although it is feasible to use image-guided IMRT to give high-dose radiation in standard fractionation, many primary tumors of the spine may respond best to high-dose hypofractionated or single-fraction radiation. However, when administering such high-dose therapy, extreme care and caution are mandatory to minimize complications. From a surgical perspective, the advantage of using highly conformal delivery of radiation is the decreased soft tissue damage and consequent decreased risk of wound complications. Patients may safely undergo surgery within 2 weeks of image-guided IMRT. The technique for spine radiosurgery36 utilizes an inverse optimized treatment planning. Typically, five to seven radiation beams are focused around a single isocenter. Each beam’s intensity is modulated with multileaf collimation using a sliding window technique similar to that for IMRT. All target volumes are generated based on International Commission on Radiation Units and Measurements (ICRU) report 50 and on the guidelines set by the International Stereotactic Radiosurgery Consortium.37 The gross tumor volume (GTV) is defined as gross disease visualized on MRI and CT imaging. The clinical target volume (CTV) was defined as the GTV as well as areas of potential microscopic spread. The planning target volume (PTV) included the CTV with a 2- to 3-mm margin for setup uncertainty and potential patient motion. In cases of epidural disease, the CTV and PTV overlap in the region of the spinal canal in order to spare the spinal cord. As part of the planning process, all volumes are reviewed by a team of radiation oncologists and neurosurgeons. Similarly, with anterior extraosseous disease around the esophagus, CTV and PTV overlap GTV to limit esophageal dose. The spinal cord, as defined on a simulation CT myelogram, is constrained to a maximum dose of 14 Gy to a single voxel, and no treatment plan should exceed this level. The esophagus is constrained to 14.5 Gy to 2.5 cc of esophagus, although this constraint can be exceeded at the discretion of the treating physician. The cauda equina is limited to maximum dose of 18 Gy. Bowel is constrained to allow no more than 5 cc to receive more than 16 Gy. In cases of significant bowel overlapping target volumes in the sacrum, saline may be infused between tumor and bowel during treatment to temporarily displace bowel away from the anterior aspect of the target volume.38 Conventional radiation treatment of sarcomas of the spine requires prolonged treatment courses and results in poor local control. Hypofractionated and single-fraction image-guided SRS (IG-SRS) may provide a more effective means of managing these radioresistant lesions. Primary sarcomas, although uncommon, carry a high mortality burden—nearly 50%.39 Metastatic disease in the spine is far more common, accounting for up to 70% of all metastases to bone and affecting up to 10% of all cancer patients.40,41 Aggressive surgical and radiotherapeutic approaches are often required to prevent tumor-associated neurologic damage, establish local control, and potentially improve overall survival.40,42 Radiation therapy is essential in spinal lesions to optimize local tumor control.43,44 Conventional external beam radiation therapy (C-EBRT) provides limited control, as spinal sarcomas are considered radioresistant.42,45,46 Yet, SRS may produce local control rates greater than 90% with low risk of injury to nearby organs and the spinal cord.40,47–49 High-dose single or hypofractionated IG-SRS has also been implemented in the postoperative setting with similarly good control rates.50–52 At MSKCC between 2005 and 2012, 147 primary (26.5%) and metastatic (73.5%) spinal sarcoma lesions were treated, 49 with hypofractionated SRS (mean 24 Gy in three fractions) and 98 with single-fraction SRS (24 Gy). Median follow-up was 14.1 months (range, 1–80.7 months); for patients with primary lesions the median follow-up was 19.1 months and for metastatic lesions the median follow-up was 12.5 months. Of the 147 lesions, 18 (12.2%) were previously irradiated; 49 (33.3%) of the lesions were treated with IG-SRS postoperatively, and 12 (8.2%) were treated with neoadjuvant SRS. Overall local progression-free survival at 12 months was 92.1% (95% confidence interval [CI], 86.9–97.3%); overall survival at 12 months was 70.7% (95% CI, 61.7–79.7%). Patients treated with single-fraction IG-SRS had a significantly better local progression-free survival than patients treated with hypofractionated IG-SRS, 88.2% (95% CI, 80.2–96.2%) versus 68.2% (95% CI, 47.8–88.6%) at 24 months (log-rank p = 0.005). Sixty-one lesions in 58 patients were treated with neoadjuvant or adjuvant IG-SRS (i.e., radiation therapy before or after surgical management); 49 lesions were irradiated postoperatively and 12 preoperatively. Overall survival and local progression-free survival at 1 year were 70.1% (95% CI, 57.5–82.7%) and 88.1% (95% CI, 78.9–97.3%), respectively. This cohort included both metastatic and primary patients; for the metastatic subset (n = 32 patients, 35 lesions), the overall survival and local progression-free survival at 1 year were 61.2% (95% CI, 43.6–78.8%) and 86.5% (95% CI, 73.9–99.1%), respectively; for patients with primary lesions (n = 26 patients, 26 lesions), overall survival and local progression-free survival at 1 year were 81.8% (95% CI, 65.4–98.2%) and 90.2% (95% CI, 77–100%), respectively. Overall survival and local progression-free survival were not significantly different (log-rank p = 0.123 and 0.442, respectively) for primary versus metastatic lesions. Timing of the radiation with respect to surgery did not have a significant effect on local progression-free survival (log-rank p = 0.170); however, patients treated with preoperative IG-SRS did have improved overall survival (log-rank p = 0.040). Patients treated with surgery and single-fraction IG-SRS had significantly better overall and local progression-free survival than patients treated with hypofractionated IG-SRS (log-rank p = 0.030 and 0.013, respectively). Eighteen lesions in 17 patients were treated with salvage IG-SRS (i.e., radiation therapy with or without surgical management for a previously treated lesion); 15 (83.3%) were treated in the postoperative setting. Overall survival and local progression-free survival at 1 year were 42% (95% CI, 14.6–69.4%) and 100%, respectively. This cohort included both metastatic and primary patients; for the metastatic subset (n = 9 patients, 10 lesions), overall survival and local progression-free survival at 1 year were 34.6% (95% CI, 0–71.2%) and 100%, respectively; for patients with primary lesions (n = 8 patients, 8 lesions), overall survival and local progression-free survival at 1 year were 50% (95% CI, 9.2–90.8%) and 100%, respectively. Overall survival and local progression-free survival were not significantly different (log-rank p = 0.197 and 1, respectively) for primary versus metastatic lesions, which reinforces the utility of SRS in the treatment of primary spinal sarcoma. DeLaney et al53 report a series of 50 patients with spinal sarcomas, of which 86% (43/50) were either chordoma or chondrosarcoma. The other seven patients were single pathologies, including osteosarcoma, Ewing’s sarcoma, giant cell sarcoma, angiosarcoma, liposarcoma, and malignant peripheral nerve sheath tumor spindle/round cell—further illustrating the challenges in differentiating the many sarcomatous histologies. DeLaney et al demonstrated 5-year actuarial local control of 78%. They delivered radiation using a “shrinking fields” technique: 50.4 CGE (Gy RBE [relative biological effectiveness]) to subclinical disease, 70.2 Gy RBE to microscopic disease in the tumor bed, and 77.4 Gy RBE to gross disease at 1.8 Gy RBE qd. The spinal cord dose was limited to 63/54 Gy RBE to surface/center. Intraoperative boost doses of 7.5 to 10 Gy could be given by dural plaque. This study found extremely high local control in 34 of 36 (94%) primary tumors and in 23 of 23 (100%) primary chordomas. This reinforces the importance of combined radiation and surgical therapy at the time of primary presentation.54 At MSKCC, Yamada et al reported a series of 14 patients with primary spine/paraspinal sarcomas treated with multifractionated stereotactic and image-guided IMRT coupled with noninvasive body frames.24 Previously unirradiated patients received a median prescribed dose of 70 Gy (range, 59.4–70 Gy) with a median planning target volume receiving the prescribed dose of 90%. The median dose maximum to the cord was 68% of the prescribed dose for previously unirradiated patients. Of the primary lesions, 81% exhibited local control with 2 to 30 months of follow-up. No cases of radiation-induced myelopathy were encountered. Chordoma is a rare and relentless disease of the skull, spine, and sacrum.55 Similar to the majority of mesenchymal tumors, it is not considered sensitive to radiation treatment. Intralesional or marginal resections in the spine and sacrum have a high probability of recurrence3; thus, en-bloc resection to achieve wide margins has been advocated as the only cure for chordomas.1 Despite major surgical advances, total en-bloc resection may only be achieved in approximately 50% of sacral chordomas, with much lower rates for lesions of the spine or skull base; therefore, recurrence is common without en-bloc resection.56,57 In part this may be due to the lack of effective adjuvant therapies.55 Radiotherapy has often been used as adjuvant as well as primary treatment for chordomas. Good control rates were achieved in some series after en-bloc excision of primary chordomas in combination with preoperative and postoperative high-dose radiation,53 yet recurrence rates in excess of 50% have been demonstrated in other series with long-term follow-up.58 Because the tolerance dose of the spinal cord, brainstem, cranial nerves, and rectum is much lower than effective doses to treat chordomas, delivery of high doses is limited. For example, treatment of the sacrococcygeal region with high doses of photon radiation therapy (45–80 Gy) can be achieved because the region is less susceptible than the cervical spine, where myelopathy due to radiation injury is common.59 Radiation doses > 70 Gy are required for gross disease at conventional fractionation schemas.60 Treatment with conventional radiation therapy at doses of 40 to 60 Gy has led to poor results, with 5-year local control of only 10 to 40%.61–64 The best reported local control rate with photons based on modern, fractionated stereotactic radiation therapy for lesions in the skull base is a 5-year actuarial rate of approximately 50%.58 Over the years, radical en-bloc surgical resection and radiation dose escalation have been further explored to improve survival. Pearlman and Friedman65 suggested a possible dose–response effect, although it has been established that increasing radiation doses in excess of 70 Gy will lead to long-term tumor control.9,66 Image-guided technology coupled with intensity-modulated photon radiation has made it possible to deliver very high dose radiotherapy to the spine and sacrum in a single fraction. A series at MSKCC showed that single-fraction spine radiosurgery can provide long-term local control in greater than 90% of cases for metastatic tumors considered resistant to conventional radiation.48 Single-session high-dose radiosurgery for skull base chordomas has shown promise.34,67 Recently, Yamada et al55 reviewed their experience with single-fraction high-dose SRS for chordoma of the spine and sacrum, and demonstrated good tumor control with low treatment-related morbidity. This series reported 24 patients with chordoma of the sacrum and mobile spine after being treated with high-dose single-fraction SRS (median dose, 24 Gy). Twenty-one primary and three metastatic tumors were treated. Seven patients were treated for postoperative tumor recurrence. In seven patients SRS was administered as planned adjuvant therapy, and in 13 patients SRS was administered as neoadjuvant therapy. Although surgery was planned in most cases where single-fraction SRS was delivered as the initial treatment, the lack of symptomatic and radiographic progression after SRS resulted in several patients refusing surgery. Thus, only six of 13 patients who underwent neoadjuvant SRS proceeded to surgery. This cohort provides information about local tumor control with single-fraction SRS as the sole treatment of newly diagnosed chordomas. The overall median follow-up was 24 months. Of the 24 patients, 23 (95%) demonstrated stable or reduced tumor burden based on serial MRI. One patient had radiographic progression of tumor 11 months after SRS. Complications were limited to one patient who developed sciatic neuropathy and one with vocal cord paralysis. The most optimistic results in the treatment of chordomas have been reported by proton therapy for the skull base, in general combining maximal surgical tumor resection with adjuvant high-dose particle therapy. The primary advantage of a proton beam is the protons’ physical feature of the Bragg peak, which provides excellent conformity of the irradiation field. Utilization of this modality has been pioneered at Massachusetts General Hospital in collaboration with the Harvard Cyclotron Laboratory. A technique called spot scanning was developed at the Paul Scherrer Institute and reported on 26 patients with extracranial chordomas and a median follow-up period of 35 months after proton therapy. In this study, a 3-year disease-free survival of 77% was documented.68 Most of the data on chordomas are taken from skull bases reports. A review of this literature demonstrates local control rates ranging from 67% at 3 years to 98% at 5 years with doses ranging from 50 to 83 Gy RBE.69–73 Because chordomas are rare and because it is difficult to randomize patients to treatments other than standard of care, it is unlikely that phase 3 trials will be done to compare different types of radiation, making the differences in clinical effect difficult to interpret.16 The use of radiotherapy as primary or adjuvant treatment for chordoma is still debated. In the past, stand-alone radiotherapy was proven to be ineffective when coupled with debulking or palliative therapy.74 It is clear that with the adjunct of single-fraction SRS and modern proton beam schemas, the role of adjuvant, neoadjuvant, and stand-alone radiotherapy will continue to evolve in the management of spinal chordomas. Chondrosarcoma is the third most common type of primary bone tumor (after myeloma and osteosarcoma) and accounts for 20 to 27% of all primary malignant osseous tumors.75 Typically these lesions are low grade and can arise de novo or from a preexisting cartilage lesion such as an osteochondroma or enchondroma. Less than 10% of all chondrosarcomas occur in the spine. Due to their biology, low percentage of dividing cells, and poor vascularity, these tumors have a tendency to be chemo- and radiotherapy resistant. However, chondrosarcomas grow slowly and rarely metastasize.76 Surgical resection remains the mainstay of treatment of chondrosarcomas. The extent of surgical resection and adjuvant therapy is dependent on the clinical and histological characteristics of the lesions. Although wide, en-bloc excision is ideal for intermediate- and high-grade chondrosarcoma, less aggressive approaches may be acceptable for low-grade chondrosarcoma. Due to their similar management challenges, chordoma and chondrosarcoma treatment series are often combined. As with chordoma management, radiation of chondrosarcoma is considered after incomplete resection, aiming at maximal local control (curative) and in situations where resection is not feasible or would cause unacceptable morbidity (palliative). For curative intentions, doses > 60 Gy are required to achieve local control.76 Due to the limitations of conventional radiotherapy, proton and photon radiotherapy in chondrosarcoma are utilized. As with chordomas, most of the data on chondrosarcoma are taken from skull base reports. The literature demonstrates local control rates ranging from 94% at 3 years to 73% at 5 years with doses ranging from 50 to 83 Gy RBE.69–73 Similar to chordoma treatment, the importance of an escalated dose is emphasized. Austin et al6 reported 26 local relapses in 141 patients treated by proton irradiation for chordoma or chondrosarcoma of the cranial base or cervical spine. Among 26 relapses, only six were reported in an area that had received 70 Gy or more versus 15 relapses in areas receiving a lower dose. Often the shielding of critical anatomic structures adjacent to tumor boundaries is the cause of underdosing and local failure.77 The skull base literature reinforces the relationship between dose inhomogeneity and risk of local failure.78 Noël et al77 demonstrated 3-year local control rates of 69% in chordoma and 91% in chondrosarcomas. Their results emphasize the importance of adequate coverage of the target volume at an “effective” dose. Another important finding by Noël et al is that the minimum dose correlates to local control, a variable that is determined by dose constraints of normal structures. Still most authors of reports in the skull base literature believe that surgery is the mainstay of treatment for this pathology.79,80 Published data demonstrate that local control is dependent on the size of the residual tumor at the time of radiation.70 Radiotherapy with carbon ions or other charged particles is another attractive radiation modality. The physical advantages of protons are combined with a higher radiobiological activity. Schulz-Ertner et al81 reported the effectiveness and toxicity of carbon ion radiotherapy in chondrosarcomas of the skull base. From available data, en-bloc resection achieving negative histological margins may have a good rate of local tumor control. For patients who are not candidates for marginal or wide resections, adjuvant radiation adds a measure of tumor control. Proton beam, intraoperative radioactive implants, and high-dose conformal photon therapy all play a role in local tumor control and possibly cure. Advances in stereotactic planning are overcoming many of the tissue tolerance issues with high-dose photon radiosurgery. The success of proton therapy depends on dosimetric factors, mainly those related to dose homogeneity within the target, rather than on the prescribed dose. However, high-quality surgical resection remains critical, because it can dramatically improve anatomic relations between tumor and adjacent anatomic structures, which is a prognostic factor of dose homogeneity.77 Pearl Pitfall
Radiation Therapy for Primary Bone Tumors
Introduction
Surgery
Radiation Therapy
Proton Beam
Intensity-Modulated Radiation Therapy
Brachytherapy
Stereotactic Radiation
Sarcoma and Radiation Treatment
Chordoma
Chondrosarcoma
Chapter Summary
Understand the importance of multidisciplinary management for optimal treatment of primary spine tumors.
Image-guided radiotherapy, including stereotactic radiosurgery, provides a level of precision of treatment delivery that enables much higher doses of radiation to be given safely to primary spine tumors.
Hypofractionation is a strategy to increase the biological effectiveness of radiation and may be an effective treatment strategy for primary spine tumors.
Intraoperative dural brachytherapy is a method of delivering high-dose radiation to the dural margin without significantly increasing the dose of radiation received by the spinal cord.
Proton beam radiation and other charged particle radiation will continue to play an important role in the management of primary spine tumors.
Patients should be referred to experienced centers for evaluation where multidisciplinary assessment is available and sufficiently high-dose radiation therapy can be provided.
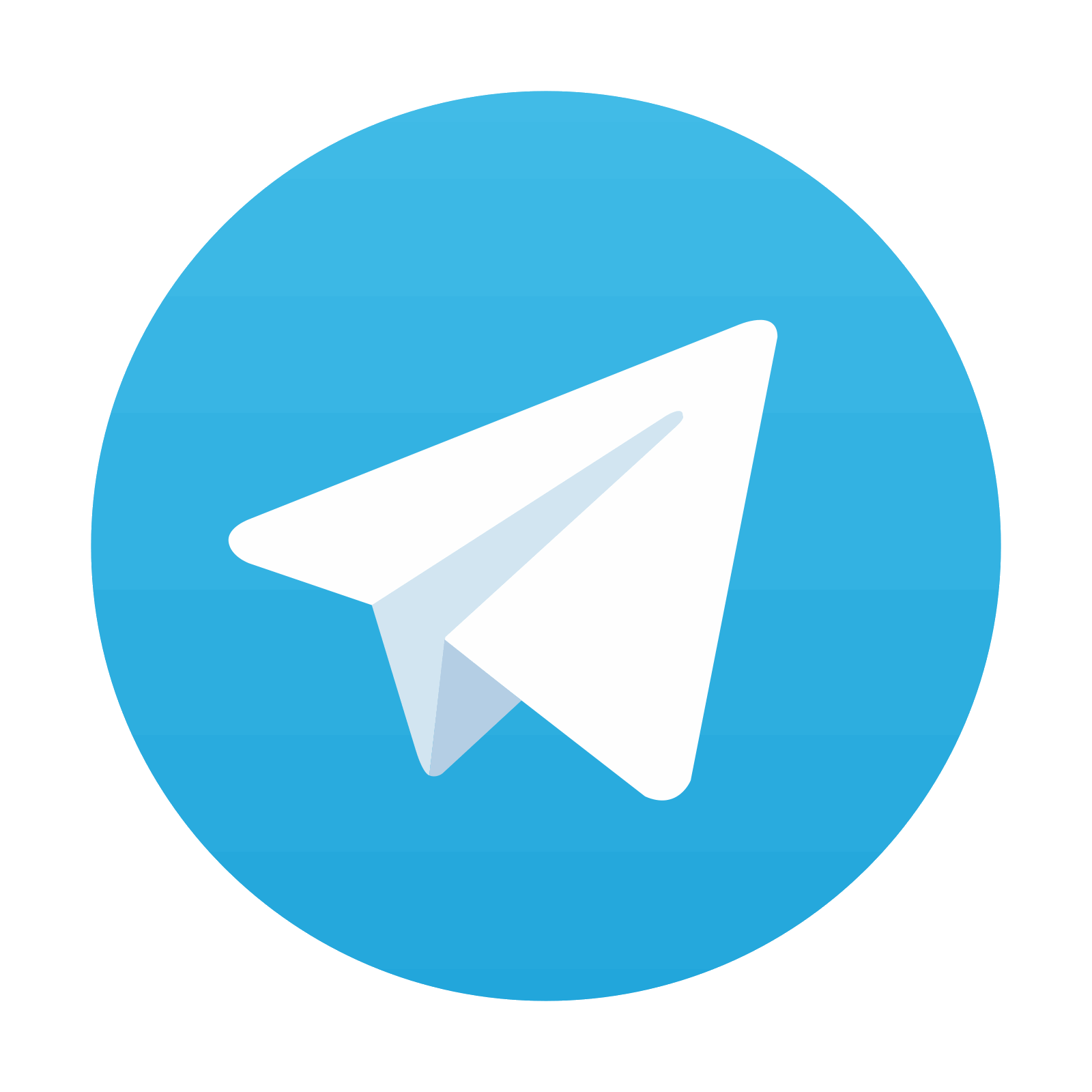