Fig. 2.1
Tissue biomechanical assessment option diagram
The purpose of this chapter is to review the current treatment options and the existing literature on outcomes, complications, and safety profile of biologic-based products and the biomechanical assessment evidence available after the utilization of these products in the literature.
2.2 Autologous Platelet-Rich Plasma
PRP has been used for more than 50 years in the dermatologic and maxillofacial fields. However, the study and application of this treatment in orthopedics is recently growing [8]. The biological rationale for the clinical use of PRP includes the local action of growth factors, the modification of the inflammatory response, and the effects on cell proliferation and differentiation [9]. PRP has been classically defined as “a volume of plasma that has a platelet count above baseline” [10]. More recent literature supports a more quantitative definition, requiring PRP to contain more than one million platelets per milliliter (mL) of serum or five times the amount of baseline platelets [11]. This elevated platelet content in PRP is necessary to effectively stimulate targeted injured cells to proliferate [12, 13]. Conversely, a recent study by Fleming et al. [14] reported that only a baseline concentration of platelets improved healing over traditional ACL reconstruction. Moreover, when the platelet concentration was increased to up to five times the baseline, it did not prove to further improve the graft mechanical properties in their study.
PRP has numerous bioactive properties that enhance cellular adhesion and regenerative capabilities including insulin growth factor-1 (IGF-1), transforming growth factor-B1 (TGF-B1), vascular endothelial growth factor (VEGF), hepatocyte growth factor (HGF), platelet-derived growth factor (PDGF), fibroblastic growth factor (FGF), and platelet factor-4 (PF-4). Other proteins such as fibronectin, vitronectin, fibrinogen, prothrombin, IGF-1, and HGF naturally concentrate in plasma and are also emitted from the α-granules upon platelet activation [15]. Certain GFs are selectively regulated by granule proteins for either GF activation or inhibition [16]. However, endogenous and exogenous mechanisms can activate platelet α-granules to secrete high concentrations of GFs, cytokines, and chemokines over a 7-day period [15].
At present, there is no consensus on the optimal preparation method and composition of PRP for each clinical indication [17]. There is no definitive evidence of the mechanism of action of PRP preparations and uncertainty that one compound could effectively treat several conditions. The real problem when trying to analyze PRP data is the great variability that exists among different products and the different responses to these treatments [18]. Low leukocyte PRP is reported to induce greater cell growth by stimulating chondrocyte anabolism, whereas leukocyte-rich PRP promoted catabolic pathways involving various cytokines [19] and can produce more side effects [20]. Dragoo et al. found that leukocyte-rich PRP treatment for tendinopathy induced early increased cellularity and new vasculature in an acute inflammatory environment [21]. Acute inflammation may be a better conductor for surrounding natural healing factors when compared to chronic tendinopathy pathologies. However, inflammation may be detrimental in the treatment of osteoarthritis or other forms of degenerative, chronic arthritis. In vitro studies have suggested a possible detrimental role of leukocytes when used in the intra-articular environment, although clinical studies are not yet conclusive [22].
The scientific justification for the use of platelets in sports medicine and orthopedic applications has gain momentum throughout the years. Platelets and plasma are biologically diverse but complimentary. Both platelets and plasma interact within supernatant due to their intrinsic GF interactions, which can be manipulated based upon exogenous or endogenous activation. GFs are versatile proteins that can activate an array of bioreactions while facilitating co-expression of one another. For example, transforming growth factor-B1 (TGF-B1) isoform coactivates osteoblast and chondrocyte generation with IGF-1 [23]. IGF-1 can also facilitate osteoblast activation with the same pathways by itself [24]. Studying GF pathophysiologic interactions in models that mimic in vivo environments is necessary to better understand how to activate certain pathways for desired tissue regeneration and repair.
There are numerous reports of PRP augmentation in reconstruction or repair interventions of the knee [25–29], shoulder [30–33], ankle [34–38], spine [39–42], and elbow [43–47]. PRP has also been applied to focal cartilage defects and osteoarthritic diarthrodial joints, such as the knee [48–54] and hip [55–57]. Favorable outcomes have been reported in treated joints, but there are also studies that have proved the contrary. The knee is the most observed joint with PRP treatment. Intra-articular PRP treatment in focal chondral defects and osteoarthritis (OA) has been shown to reduce pain while improving range of motion and quality of life. In most clinical observations, PRP is only beneficial for a short period of time [49]. A recent systematic review by Campbell assessed clinically relevant improvements after PRP treatment in cartilage defects and found that intra-articular PRP injection is a viable modality up to 12 months in early-stage OA [58]. Campbell also observed a local adverse reaction after multiple PRP injections [58].
Currently, the recommended dosage or frequency of PRP treatment or on the type of PRP that should be used for intra-articular treatment remains unclear. Cole et al. conducted a systematic review on all RCTs that studied the clinical effects of leukocyte-poor and leukocyte-rich PRPs versus hyaluronic acid for the treatment of knee osteoarthritis [59]. Three RCTs that used leukocyte-poor PRP reported positive outcomes compared to hyaluronic acid, while only one RCT using leukocyte-rich PRP reported positive effects versus hyaluronic acid [59]. Several studies have reported pain reduction, functional improvement, and reduced prevalence of surgical revisions and arthrofibrosis, but further basic science evidence is necessary to determine the effects of leukocyte-poor or leukocyte-rich PRP for intra-articular treatment. The effects of PRP treatment on in vivo ligament healing have been most commonly studied in the anterior cruciate ligament (ACL). Some studies have reported improvement in ACL healing when biomechanical, MRI, or pain assessment outcomes have been measured [60–62]. Conversely, other studies report no beneficial effects on ACL healing when these same parameters have been analyzed [63, 64]. Conflicting reports on the potential beneficial effects of PRP on healing damaged ligaments besides the ACL, coupled with the lack of basic science research, have left a large gap in evidence that is necessary to justify the use of this commonly sought-after treatment.
In summary, although several clinical studies have reported improvements in patient-reported outcomes (PROs) and significant reduction in pain scores following PRP treatment in damaged tissue [65], there is a paucity of literature that has consistently used a standard methodology to process and activate these PRP resultants, making it very difficult to reproduce similar clinical results after PRP therapy or compare the effects of PRP on various musculoskeletal conditions between studies.
2.3 Bone Marrow Aspirate Concentrate
BMAC has emerged as a significant biological option for the orthopedic surgeon because it is one of the few methods of delivering progenitor cells and growth factors currently approved by the US Food and Drug Administration (FDA). However, it has been reported that in bone marrow aspirates, mesenchymal stem cells only represent 0.001–0.01% of mononuclear cells after density gradient centrifugation to remove red blood cells, granulocytes, immature myeloid precursors, and platelets [66, 67]. However, BMAC also offers a rich source of growth factors, which may synergistically contribute to chondrogenesis, but also its anabolic and anti-inflammatory effect [68]. An important component of BMAC isinterleukin-1 receptor antagonist (IL-1 RA) (inhibits IL-1 catabolism). Cassano et al. [69] reported that BMAC has a significantly greater amount of monocytes and IL-1 RA, which is thought to be responsible for the early beneficial effects of the biologic autologous conditioned serum [70].
BMAC has a variety of therapeutic applications within the literature. These applications range from surgical injection augmentation, scaffolding augmentation, and conservative injection therapy. In a recent study, Achilles rupture repair following BMAC injection resulted in early mobilization and zero secondary ruptures over a 2-year period compared to the untreated group [71]. However, an in vitro histological observation of the ACL graft integration site resulted in nonsignificant differences between the treated and untreated groups at 4 and 8 weeks [72]. In a systematic review, 11 studies that observed knee OA (n = 8) and focal cartilage defects (n = 3) treated with BMAC reported good to excellent patient-reported results [73]. The authors concluded that BMAC appears to be a safe procedure with good to excellent reported results. However, the studies used different processing techniques, indications, and outcome measures, and this heterogeneity does not allow to draw any conclusions [73].
The outcome disparities between PRP and BMAC pose the question of which musculoskeletal condition would benefit from one biotherapy over the other. Moreover, to improve biologic quality for surgical applications, further observations are needed to elucidate the bioactivity and results between BMAC-derived factors and scaffolds. Several studies have suggested that BMAC improves patient-reported outcomes and quality of life and restores cartilage formation and function, but further randomized human clinical trials and basic science analyses are needed to clarify the efficacy of BMC therapy (Fig. 2.2).


Fig. 2.2
Picture demonstrating bone marrow aspiration from a right posterosuperior iliac spine (PSIS). The trochar is inserted into the cancellous bone of the iliac crest after penetrating the cortical bone with the use of a power drill and the sample is obtained
2.4 Cell-Based Therapies
Progenitor cells include any cell that can proliferate to form progeny and can differentiate into a derived tissue. Stem cells are a special subset of progenitor cells, which have “self-renewal capacity” [74–78]. Self-renewal is the process where a cell divides asymmetrically, producing two daughter cells. The first daughter cell is identical to the initial cell and remains available for another asymmetrical “self-renewing” cell division. The second cell is a progenitor cell which, unlike the stem cell, proceeds to divide and differentiate. Progenitor cells are far more prevalent than stem cells in any tissue. Often the term “stem cell” is used incorrectly to describe both stem and progenitor cells as a whole [79, 80].
The use of an accurate standardized nomenclature is crucial for understanding the biological behavior of cells in vivo and in vitro and improves science communication. Stem cells can be classified into several ways: (1) autologous or allogeneic; (2) adult, embryonic, or IPSCs (induced pluripotent stem cells); and (3) native (tissue resident) or culture expanded.
Many terms have been used to describe the same adult stem and progenitor cell populations in the native tissue. In an approach to provide clarification, the term connective tissue progenitors (CTPs) has been proposed [81]. CTPs include the entire heterogeneous native (tissue resident) population of stem and progenitor cells, with the potential to be activated and generate progeny that can contribute to one or more connective tissues (e.g., bone, fat, cartilage, fibrous tissue, blood, and muscle) [74, 75, 82]. CTPs are resident in and can be harvested from the bone marrow, fat, cartilage, and other tissues. However, CTPs in each tissue often have different niches, biological attributes, and potential. The term CTP recognizes that these tissue-derived cells are not a uniform population, and until detailed characterization is achieved, CTPs may only be detectable by their capacity to proliferate and form colony on a 2D surface or in a 3D viscous medium colony forming unit (CFU) assay [74, 75, 82].
Assessing single-cell mechanics has led to a better understanding of the mechanisms that govern chondrocyte/tenocyte/muscle cell mechanobiology. By modeling the biological responses to cellular deformation, a deeper understanding of the cell responses to loading and mechano-transduction at the single-cell level has been developed. A thorough knowledge of mechano-transduction at the single-cell level can provide the basis for the selection of appropriate stimuli for tissue engineering.
Cytodetachment quantifies cell adherence. These methods were developed to quantify the force required to displace attached cells. It has been shown that cellular adhesion plays an important role in embryonic development, which is significant for tissue engineering applications that often attempt to recapitulate development. By quantifying the force required to displace cells from each substrate, it was shown how cells adhere differently to different materials. This work has widespread implications because many tissue engineering techniques involve culturing cells on substrate materials.
Cytocompression alters gene expression and deforms nuclei. Compressing chondrocytes have been shown to influence gene expression, potentially due to nuclear deformation. For instance, statically compressing chondrocytes were found to modulate gene expression of extracellular matrix (ECM) proteins in a dose-dependent manner. Increased force exposure catabolically shifted single-cell mRNA levels of aggrecan, collagen type II, and tissue inhibitor of metalloproteinase-1. This work showed that single cells respond to static compressive force by modifying gene expression related to ECM synthesis and maintenance.
2.5 Biologics and Biomechanics
As the amount of research directed toward biologics and synthetic biologic products has expanded, there has been a growing effort to characterize and define the ideal applications for orthobiologics. Biomechanical evaluation is a key factor to evaluate and further improve biological approaches.
2.5.1 Articular Cartilage Regeneration
Articular cartilage degeneration can occur due to injury, aging, or both. Repetitive microtrauma, instability, or undetected intra- or extra-articular injuries may lead to an accelerated degeneration of the joint surface. This process can occur rapidly or over the course of several decades. Because of the growing number of patients afflicted with osteoarthritis at increasingly younger ages, more attention has been directed toward tissue engineering techniques to disrupt or reverse the osteoarthritis development process [83]. A recent biomechanical study reported on the suture retention strength of in vitro prepared neocartilage. The authors found that neocartilage had 33% of the tensile strength of native cartilage. This study also reported survival of neocartilage grafts sutured into osteochondral defect rabbits. The authors concluded that neocartilage can be reliably secured with sutures [84]. Additional biomechanical studies have demonstrated early arthritic changes in adjacent tissues in patients with suture-secured cartilage implants [85]. However, a recent in vitro study of nanoindentation in repair and native cartilage revealed that repair cartilage had a ten times lower contact stiffness than that of native cartilage [86]. Other biomechanical studies have also reported decreased contact stiffness and modulus in repair tissues compared to native articular cartilage [87]. Although techniques to produce neocartilage have advanced, there is still further research needed to develop tissue more similar to that of native articular cartilage.
Many surgeons currently hold microfracture as the gold standard procedure in cartilage restoration for small lesions (less than 1.5 cm2) [88]. However, recent studies have indicated that although microfracture delays cartilage degeneration at short-term follow-up, the beneficial effects may not last beyond 5 years after surgery [89, 90]. Moreover, studies have reported that treatment failure after microfracture is possible regardless of osteochondral defect size [91, 92]. As the shortcomings of microfracture have been elucidated, there has been growing interest in developing treatments with the capacity to fully restore articular cartilage at extended follow-up intervals. In this regard, a recent study reported that repair of chondral injury using a hyaluronic acid-based scaffold with activated bone marrow aspirate concentrate provides better clinical outcomes and more durable cartilage repair at medium-term follow-up compared with microfracture [93].
Gobbi et al. investigated the clinical outcome in a group of active patients with large full-thickness chondral defects of the knee treated with one-step surgery using bone marrow-derived MSCs and a second-generation matrix. The authors reported that MRI scans showed good stability of the implant and complete filling of the defect in 80% of patients, and hyaline-like cartilage was found in the histological analysis of the biopsied tissue at a minimum of 3 years. No adverse reactions or postoperative complications were noted [94].
Autologous chondrocyte implantation (ACI) has developed into a promising new frontier for cartilage restoration, with potentially more consistent results than microfracture. Case series with >10 years follow-up have shown ACI to be effective and biomechanically durable for large (>4 cm2) chondral defects [95, 96]. Peterson et al. evaluated indentation measurements at the central part of each ACI graft and reported a range of 0.3–3.7, which was 90% or more of the value observed in the control group [95]. Gooding et al. [97] recently compared ACI to osteochondral transplantation at 10 years follow-up and reported superior outcomes in the ACI group compared to the osteochondral transplant group. Similar findings have been reported in other studies reporting on patients with >3 cm2 osteochondral lesions [98, 99]. These studies have increased the confidence in ACI as a reliable cartilage regeneration tool; however, further studies are needed to identify the specific indications for ACI and the long-term intra-articular biomechanical benefits of ACI. One of the major drawbacks of ACI is the need for a biological matrix to ensure correct placement of ACI in the joint being treated. Some studies have reported using porcine membrane with mixtures of type I and II collagen and/or hyaluronic acid scaffolds [97, 100, 101]. However, using such grafts may expose the patient to developing an immune reaction and have a subsequent failure of the ACI procedure. The use of matrices in ACI is necessary; however, future studies should focus on developing non-immunogenic and biomechanically stable ACI matrices.
Scaffold-based chondrocyte implantation has also emerged as a promising treatment for osteochondral defects. Hyaluronic acid-based scaffolds have been shown to facilitate development of hyaline-like cartilage 1 year after implantation in early case series [101, 102]. In a recent prospective cohort study, Kon et al. reported comparable patient-reported outcomes between a group that received a hyaluronic acid-based matrix-induced autologous chondrocyte implantation (MACI) or microfracture [102]. However, at 7.5 years follow-up, the hyaluronic acid-based MACI patients had significantly better IKDC scores than the microfracture group. The authors who also performed a sub-analysis of return to sport in the athletes in their cohort found that microfracture required 8 months of recovery, whereas the hyaluronic acid scaffold plus MACI patients required 12.5 months of recovery [102]. Gobbi et al. compared the clinical outcomes of two similar groups of patients with patellofemoral full-thickness cartilage lesions, treated with MACI or BMAC, employing the same scaffold [103]. Both groups showed significant improvement in all scores, from preoperative to final follow-up (P = 0.001), but there was no significant difference in improvement between the two groups, except for the IKDC subjective score (P = 0.015), which favored the BMAC group. Deterioration in MACI and improvement in BMAC group scores were noticed, from 2 years to final follow-up, but were nonsignificant [103]. Given the longer rehabilitation period of the scaffold plus MACI group, future studies must focus on decreasing rehabilitation times while maintaining biomechanical viability of MACI treatment.
Scaffold-free constructs also offer an option for cartilage replacement. These options are prepared by culturing bovine chondrocytes in high-density molds over 8 weeks. By week 1, these constructs could be handled [104], and by week 4, they had biomechanical properties similar to that of juvenile cartilage [105]. However, other biomechanical studies have demonstrated that cartilage repair tissue to have more rapid cyclic compression values compared to normal articular cartilage. Although scaffold-free constructs reduce the risk of immunologic reaction at the time of implantation, further studies are needed to ensure the scaffolds can withstand the biomechanics of the joint that is implanted into.
The future of cartilage regeneration lies within the realm of in vitro chondrocyte-seeded scaffold development. This process involves the introduction of chondrocytes into a 3D matrix, which is subsequently cultured in vitro for 4–6 weeks [106]. This process produces neotissue that has produced promising short- and long-term patient-reported outcome results [107–109]. Because walking exposes articular cartilage to force equal to 4–5 times a person’s body weight [110], in vitro development chondrocyte-seeded scaffolds may not produce biomechanically robust constructs. Because of this, researchers have begun to expose cell-laden matrices to hydrostatic pressure [107] or dynamic compression [108] to enhance matrix maturity and biomechanical strength. In a phase I FDA clinical trial, eight patients received autologous chondrocyte-implanted collagen I scaffolds, which were subjected to hydrostatic pressure prior to implantation. At 1 year after surgery, seven of the eight patients had almost completely filled chondral defects, with mature repair tissues [111]. In the subsequent phase II trial, the scaffold technique was found to have a similar safety profile to microfracture, but was found to have better clinical outcome scores at 2 years follow-up.
Tissue engineering has demonstrated a distinct ability for cartilage regeneration that could potentially advance the field of treating both focal chondral lesions and OA by providing a functional biological equivalent tissue to replacing lost or damaged tissue. The standard concept of tissue engineering is to seed cells on three-dimensional (3D) biomaterial scaffolds to help regenerate damaged tissue. The scaffold is designed to create a 3D environment that promotes tissue regeneration through the cells that are seeded within the scaffold [112, 113]. Multiple scaffolds have been proposed for tissue engineering. Among these, Shimomura et al. compared ahydroxyapatite (HA)-based artificial bone coupled with a mesenchymal stem cell (MSC)-based scaffold-free tissue-engineered construct (TEC) with a beta-tricalcium phosphate (bTCP) for repair of osteochondral defects in a rabbit animal model. The authors found that osteochondral defects treated with the TEC/bTCP implants showed more rapid subchondral bone repair at 1 month, but the cartilaginous tissue deteriorated over time out to 6 months after implantation. Osteochondral defects treated with the TEC/HA implants maintained good histological quality out to 6 months after implantation and also exhibited better biomechanical properties at 6 months as compared with the TEC/bTCP implants [114].
Furthermore, biodegradable hydrogels have been suggested as a promising scaffold for articular cartilage tissue engineering. A main advantage of using hydrogels is the ability to inject the hydrogel as a solution in situ and then polymerizing it in vivo. In addition, recent advances in bioprinting have granted tissue engineers the ability to assemble hydrogels into anatomically relevant functional tissues or organ parts. Moreover, hydrogels could also be potentially used as drug delivery systems, allowing a controlled and sustained release of drugs intra-articularly over several weeks or months [115] for the treatment of joint disease such as OA or rheumatoid arthritis (Fig. 2.3).


Fig. 2.3
Osteochondral defect performed in a left knee of a rabbit model. A critical size defect of 3 mm in the trochlea was treated with a hydrogel seeded with mesenchymal stem cells and photopolymerized in the defect area. The final result is demonstrated in a (a) histological cut and in the (b) necropsy examination
The body of the literature concerning cartilage tissue engineering in animal models is rapidly expanding; however, it has been reported that 90% of the new approaches that are successful in animal studies subsequently fail clinical trials [116]. Therefore, meticulous analysis of the existing short-term clinical outcomes is advocated to be able to guide the mixed biomimetic and bioactive approach to more successful and reliable clinical outcomes.
2.5.2 Rotator Cuff Augmentation and Repair
Much of the orthobiologics biomechanical literature has focused on strengthening partially torn rotator cuffs or enhancing rotator cuff repairs. Early studies focused on defining an ideal scaffold to enhance rotator cuff repair strength. Barber et al. [117] proposed a dermal allograft augmentation to strengthen supraspinatus tears. Using ten pairs of human cadaveric samples, they cyclically tested augmented and non-augmented repairs at 10 and 100 N for ten cycles at 20 N/S, followed by destructive testing at 33 mm/S. Bio-mechanical evaluation revealed a significantly greater failure load in the augmented repair shoulders. Furthermore, the two groups had different failure mechanisms: the non-augmented repairs failed at the tendon-suture anchor interface while augmented repairs failed via suture breakage. Moffat et al. [118] engineered a poly(lactide-co-glycolide) [PLGA] nanofiber scaffold to improve rotator cuff repair strength by guiding fibroblast attachment and fiber orientation. Biomechanical analysis revealed that cell distribution and nanofiber organization over the PLGA scaffold were biomechanically superior to those outside of the scaffold area, despite the fact that the PLGA scaffold degraded during in vitro testing. In a later study, Derwin et al. studied eight adult dogs that underwent bilateral shoulder surgery: one shoulder underwent tendon release and repair, while the other shoulder underwent release and augmentation [119]. At time zero, the repair augmentation shoulder had significantly higher failure load. At 12 weeks, the poly-l-lactide scaffold group had significantly greater cross-sectional area, stiffness, and ultimate load than repairs without augmentation. The authors concluded that although using a scaffold augmentation provides a medium for host tissue deposition and ingrowth, it does not fully prevent tendon retraction [119].
Further studies have demonstrated the biomechanical properties of extracellular (ECM) rotator cuff repair augmentation patches to be inferior to that of the native rotator cuff [120]. However, many techniques to reproduce an ECM with properties similar to that of the native rotator cuff have also been described [121–124]. In animal models, rotator augmentation with a porcine intestine patch demonstrated superior stiffness compared to traditional repair but failed to show an increased load to failure [125]. Interestingly, most porcine intestine patch studies report that the graft is weakest at 1–2 weeks after surgery, but increases to strength similar to native rotator cuff tendon by 3 months [126, 127]. Unfortunately, these promising findings produced in the porcine intestine patch models have not scaled into human models. Scalmbert et al. reported MRI confirmed failure in 10 of 11 patients following porcine patch placement for large rotator cuff tears [128].
Cytokine-based therapy has also been proposed and evaluated to enhance rotator cuff strength. Using a sheep model, Rodeo et al. [129] introduced BMP-(2–7) and TGF-(b1–3) in addition to fibroblast growth factor into a type I collagen sponge at the rotator cuff tendinous insertion. At 6 and 12 weeks after surgery, a higher failure load was found in the cytokine plus collagen scaffold group compared to the traditional reconstruction group. However, when these findings were normalized based on tissue volume, no significant differences were found. This study elucidated that although cytokine therapy may accelerate healing, it may not have the capacity to increase rotator cuff reconstruction strength.
2.5.3 Meniscus Transplant
Currently, there are two commercially available partial meniscus substitutes: one is made up of type I collagen fibers from bovine Achilles tendon and the other is made up of polyurethane polymers with interdigitating polyester and semi-degradable stiff segments. Early studies on the type I collagen-based implants in humans demonstrated no adverse effects, formation of new tissue, and good clinical outcome scores at 36 months [130]. A later study compared patient outcomes between patients treated with the collagen-based meniscus substitute and patients who underwent partial meniscectomy. This study reported superior patient outcomes in the patients treated with the collagen-based meniscus substitute [131]. In contrast, Rodkey et al. reported that 311 patients with Outerbridge scores less than grade IV were either treated with the collagen-based meniscus substitutive or partial meniscectomy [132]. This study reported that the collagen-based meniscus replacement resulted in improved clinical outcomes in patients with medial meniscus defects, but was not effective for patients with acute chondral injuries or lateral meniscal defects [132]. Despite promising clinical findings, magnetic resonance imaging (MRI) studies have revealed several salient biomechanical changes in the collagen-based meniscus substitutes. The size of the meniscus substitute reduces significantly during follow-up interval, and the MRI signal intensity of meniscus substitutes does not match that of the native meniscus at any follow-up periods [133, 134]. These findings indicate that although clinical results have been encouraging, further studies need to evaluate the biomechanical integrity of meniscus substitutes so that patients can have sustained outcomes beyond 10 years.
Regarding total meniscectomized patients, allograft transplantation remains the gold standard for symptomatic patients [135]. However, meniscal allograft transplants have been observed to undergo collagen remodeling [136] and shrinkage [137], which may lead to alteration of biomechanical properties. Because of these limitations, attention has been directed toward developing a synthetic total meniscus substitute. Early research focused on the biomechanical viability of Teflon and Dacron as meniscus substitutes [138–140]. However, these designs were limited by a lack of biocompatible materials and poor biomechanical performance. Later studies reported on a porous polyurethane scaffold as a total meniscus substitute. Pore size and compressive properties were tuned to stimulate local tissue growth and differentiation into fibrocartilaginous tissue, based on previous studies [141, 142].Compressive properties of the implant increased 24 months after implantation and were not different from native meniscus properties [143]. However, the meniscus implant was not strong enough to resist shear forces in the knee, and collagen type and orientation were not meniscus-like upon further evaluation [143]. Although there has been promising clinical and biomechanical studies reporting on meniscus substitutes, clinicians must remain focused on ensuring that these implants can withstand the stresses of daily life and/or athletic competition so that patients can remain symptom-free over a longer period of time.
Conclusions
Although biological approaches are rapidly evolving, limited literature is accompanying this development. Few randomized clinical trials exist regarding these therapies, and therefore there exists uncertainty on the real outcomes, safety profile, and long-term results. Promising results have been reported in the literature; however, the heterogeneity in indications, techniques, and outcome measures challenges the evaluation and comparison of outcomes. We encourage performing high-quality research with long-term follow-up to elucidate the effects of these approaches and ultimately build a solid base for the use of biological therapies in the orthopedic field.
References
1.
Mithoefer K, McAdams T, Williams RJ, Kreuz PC, Mandelbaum BR. Clinical efficacy of the microfracture technique for articular cartilage repair in the knee: an evidence-based systematic analysis. Am J Sports Med. 2009;37(10):2053–63. doi:10.1177/0363546508328414.PubMed
2.
Jorgensen C, Noel D. Mesenchymal stem cells in osteoarticular diseases. Regen Med. 2011;6(6 Suppl):44–51. doi:10.2217/rme1180.PubMed
3.
Jorgensen C, Djouad F, Bouffi C, Mrugala D, Noel D. Multipotent mesenchymal stromal cells in articular diseases. Best Pract Res Clin Rheumatol. 2008;22(2):269–84. doi:10.1016/jberh200801005.PubMed
4.
Yelin E, Weinstein S, King T. The burden of musculoskeletal diseases in the United States. Semin Arthritis Rheum. 2016; doi:10.1016/jsemarthrit201607013.PubMed
5.
Hootman JM, Helmick CG. Projections of US prevalence of arthritis and associated activity limitations. Arthritis Rheum. 2006;54(1):226–9. doi:10.1002/art21562.PubMed
6.
Sugaya K. Potential use of stem cells in neuroreplacement therapies for neurodegenerative diseases. Int Rev Cytol. 2003;228:1–30.PubMed
7.
Co C, Vickaryous MK, Koch TG. Membrane culture and reduced oxygen tension enhances cartilage matrix formation from equine cord blood mesenchymal stromal cells in vitro. Osteoarthr Cartil. 2014;22(3):472–80. doi:10.1016/jjoca201312021.PubMed
8.
Hsu WK, Mishra A, Rodeo SR, Fu F, Terry MA, Randelli P, et al. Platelet-rich plasma in orthopaedic applications: evidence-based recommendations for treatment. J Am Acad Orthop Surg. 2013;21(12):739–48. doi:10.5435/jaaos-21-12-739.PubMed
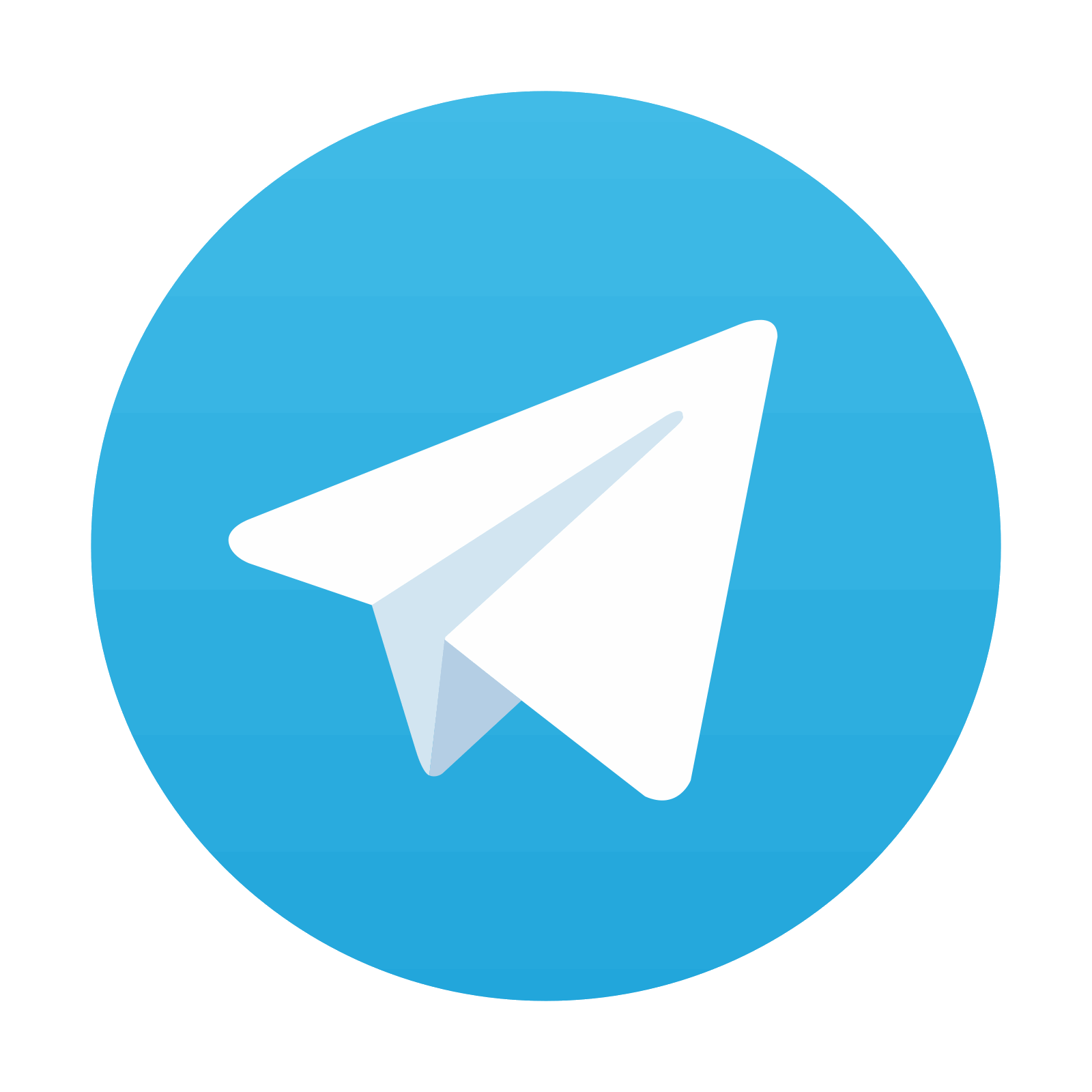
Stay updated, free articles. Join our Telegram channel
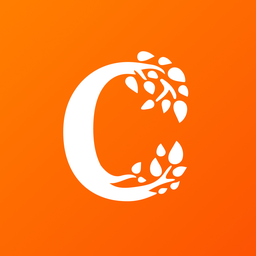
Full access? Get Clinical Tree
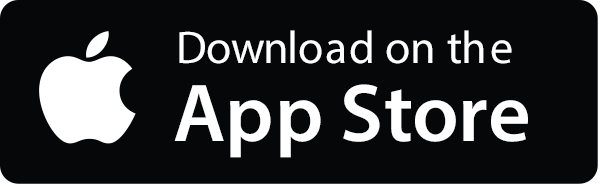
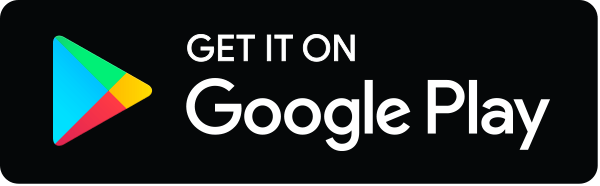