© ISAKOS 2017
Gian Luigi Canata, Pieter d’Hooghe and Kenneth J. Hunt (eds.)Muscle and Tendon Injuries10.1007/978-3-662-54184-5_55. Muscle-Tendon Junction Injury
(1)
Department of Orthopaedic Surgery, Duke University, Durham, NC 27708, USA
(2)
Michael W. Krzyzewski Human Performance Laboratory (K-Lab), Department of Orthopaedic Surgery, Duke University, Durham, NC 27708, USA
Keywords
MuscleMuscle-tendon junctionInjuryStrain5.1 Introduction
Muscle strain injury is one of the most frequently occurring and debilitating injuries in sport and recreation (Glick 1980; Krejci and Koch 1979; Nikolaou et al. 1987; O’Donoghue 1984; Ryan 1969). Both partial and full muscle strain injuries result in significant short-term and long-term consequences (Garrett et al. 1988). These consequences include time lost from work at significant compensation costs and inability to regain pre-injury levels of performance (Garrett et al. 1988). Despite having knowledge of the consequences, the understanding of muscle and tendon injuries is still vastly unknown. The exact anatomical location of damage within the muscle-tendon junction and the predisposing factors for damage in normal, healthy muscle are still unanswered questions surrounding muscle strain injury (Garrett et al. 1988). Our understanding of muscle and tendon injuries has expanded considerably in the last 30 years. Much more is known about the pathophysiology of injury in animal model and in clinical practice. However, much remains to be determined about prevention and treatment.
5.2 Structure
The structural link between the myofilaments and the connective tissues is the muscle-tendon junction (Garrett et al. 1988). The muscle-tendon junction is responsible for aiding in the transmission of tension within the muscle (Garrett et al. 1988). The tendon fibrils insert on the muscle fibers through an elaborate system of folds which increases their surface area (Schwarzacher 1960). The muscle-tendon junction, otherwise referred to as the connecting domain, has a vast number of fine filaments which are oriented approximately perpendicular to the major force vector (Trotter et al. 1981, 1983). These fine filaments connect the terminal myofibrils of the lamina densa to the collagen fibrils of the tendon (Trotter et al. 1981, 1983). These filaments have a diameter of two to seven nanometers (Ajiri et al. 1978; Hanak and Bock 1971; Korneliussen 1973). New sarcomeres are developed at the muscle-tendon junction with growth and development and/or hypertrophy as a result of stretch (Williams and Goldspink 1971, 1973, 1978). The ability to develop new sarcomeres and the plasticity at the terminal sarcomeres, located within the muscle fibers, demonstrate the available plasticity of the skeletal muscle (Williams and Goldspink 1971, 1973, 1978). The plasticity allows for localized change within the muscle-tendon junction, in addition to the possibility of regional structural and functional specializations (Garrett et al. 1988).
Important in the overall structure and function of the muscle-tendon unit are the tendon and ligament. Previous studies have examined the viscoelastic characteristics of tendons and ligaments (Butler et al. 1978; Solveborn 1983). When a tendon or a muscle is loaded with a constant force, the length slowly increases without increase in a time-dependent (therefore viscoelastic) manner (Solveborn 1983; Butler et al. 1978). Elastic structures produce a constant deformation for a constant load (Butler et al. 1978). The length-tension relation changes with load and therefore displays viscoelastic behavior rather than strict elastic behavior (Butler et al. 1978). Increased length in response to a constant force is called creep (Butler et al. 1978). If a muscle or tendon is stretched to a constant length, the measured tension decreases (Butler et al. 1978). The decrease in tension over time is also referred to as stretch relaxation (Solveborn 1983; Butler et al. 1978). Cyclic stretching of the ligament and tendon to the same length results in a decrease in tension with each stretch performed (Butler et al. 1978; Solveborn 1983). Descriptions of the muscle-tendon unit under two types of cyclic repetitive stretching have been provided by previous research (Taylor et al. 1985b, 1990). With repetitive cyclic stretching to the same length, there is an 80% increase in total length during the first four stretches (Taylor et al. 1985b). The stretch relaxation curve following the first stretch is significantly different than the stretch relaxation curve following the second stretch (Taylor et al. 1985b). The third and fourth stretch relaxation curves are also different, but the final six curves demonstrate no differences between them (Taylor et al. 1985b, 1990). Another study, similar to the previously described study, demonstrated that the peak tension decreased with each stretch, in addition to an overall drop in peak tension of 16.6% between the first and tenth stretch (Taylor et al. 1985c). There is a significant decrease in peak tension between the first four stretches, but the peak tension in cycles five through ten do not differ (Taylor et al. 1985c). This information suggests that repetitive stretching will lead to a reduction of load on the muscle-tendon junction at a given length (Taylor et al. 1985c). This effect is independent of any reflex effects or other influences mediated by the central nervous system; reflex effect and central nervous system influences may be involved in addition to the viscoelastic response (Taylor et al. 1985a).
The muscle-tendon unit is viscoelastic (Taylor et al. 1990). The decline in peak tension, which occurs because of the viscoelastic property of stretch relaxation, is associated with the internal changes in the structure of the muscle (Taylor et al. 1990). With each stretch, the relaxation curve for the muscle-tendon unit gradually levels off at higher tensions than the preceding relaxation curve (Taylor et al. 1990). Although a constant amount of tension is not always maintained to cause a length increase, varying amounts of tension up to a maximum lead to elongation (Taylor et al. 1990). This is demonstrative of the creep property of the muscle-tendon junction, forming a curve toward a maximum deformation (Taylor et al. 1990). Both peak tensile force and absorbed energy are dependent upon the rate of stretch applied (Taylor et al. 1990). Stretch rate dependency is the amount of stress relaxation that occurs in a given amount of time (Taylor et al. 1990). Slower stretches allow for a greater degree of stretch relaxation to occur, resulting in lowered peak forces (Taylor et al. 1990). Energy is absorbed by the muscle-tendon unit during the loading process and is then dissipated during the unloading process (Taylor et al. 1990). During any one stretch, the rate at which the muscle-tendon unit absorbs energy is different from the rate at which it dissipates energy (Taylor et al. 1990). This creates a discrepancy between energy put into the system and the energy released form the system (Taylor et al. 1990). This difference may be explained by heat transfer and/or by internal changes within the ultrastructure of the muscle (Taylor et al. 1990).
5.3 Function
The function of the muscle and muscle-tendon junction has implications for muscle strain injury. The amount of stretch that a muscle may endure impacts the likelihood of having a muscle strain injury (Garrett 1990). Muscles that act on two joints are subjected to more stretch than muscles that act on one joint and are, therefore, more likely to suffer muscle strain injuries (Brewer 1960). The ability to be stretched at more than one joint, based on anatomical location, may play a role in the risk of muscle strain injury associated with these two joint muscles (Brewer 1960; Garrett 1990). These two joint muscles have an intrinsic tightness; in turn they can limit the ability to produce range of motion, but normal physiological joint motion can place the muscles in a position of increased passive tension (Brewer 1960; Garrett 1990). Often times these types of muscles control the regular movement when they are eccentrically contracted (Brewer 1960; Garrett 1990). More specifically, they act to produce joint motion control or decelerate the joint, both actions that are eccentric in nature (Brewer 1960; Garrett 1990).
Normal muscle activated by way of nerve stimulation, which has been previously theorized to lead to muscle strain injury, does not cause complete or incomplete disruption of the muscle (Garrett et al. 1984a). Although force is diminished and there is a failure of the excitation, there is no disruption of the muscle and no muscle strain injury (Garrett et al. 1984a). In order to see any gross microscopic muscle injury, stretch of the muscle is required, as opposed to solely nerve stimulation of the muscle (Garrett et al. 1984a). At the time of muscle failure, the forces produced without muscle activation are several times higher than the maximum isometric force produced by the activated muscles (Garrett et al. 1988). In turn, the passive forces within the muscle may play as much of a role as the active forces that occur in the muscle during muscle strain injury (Garrett et al. 1988).
The passive components of a stretched muscle have the ability to absorb energy, but that potential is greatly increased by active contraction of the muscle (Garrett 1990). This may provide an explanation as to why muscles can be injured when they are incapable of withstanding strain (Garrett 1990). A muscle’s ability to withstand strain is a measure of the energy absorbed by the muscle prior to failure (Garrett 1990). Strain energy is represented as the area underneath the curve as it relates stress to strain (Garrett 1990). The two components that compose a muscle’s ability to absorb energy include the passive component which is not dependent on the muscle activation and is a property of the connective tissue elements within the muscle, including muscle fiber and the connective tissue in the cell surface and existing between the fibers (Garrett 1990). There is an additional ability to absorb energy based on the contractile ability of the muscle (Garrett 1990). The active muscle components can double the ability of a muscle to absorb energy; therefore, conditions which diminish the ability of a muscle to contract might also diminish the ability of the muscle to absorb energy (Garrett 1990). Muscle fatigue and weakness are considered predisposing factors for muscle injury; this implies that the active ability to absorb the energy is diminished (Garrett 1990). The ability of a muscle to absorb energy can protect the bone and joint, as well as the muscle itself (Radin et al. 1979). When the muscle is under a low strain, the energy absorption is due to the active component as opposed to the passive component (Radin et al. 1979).
5.4 Injury
A muscle strain is the response of a muscle following forceful stretching in an active or passive manner (Garrett 1990). Muscle strains may be partial or complete (Garrett 1990). These injuries are typically acute and usually a painful event, which is recognized by the patient as the event of injury (Garrett 1990). Two types of muscle strain injuries are possible, direct injury and indirect injury (Garrett 1990). Direct muscle strain injury is characterized by a contusion, which in turn causes an injury based on direct contact to a portion of the muscle (Garrett 1990). An indirect injury is typically at or near the muscle-tendon junction or the tendon-bone junction (Garrett 1990). A complete tear indicates that the muscle is asymmetric when compared to the non-injured contralateral side when the patient is at rest (Garrett 1990). When a complete tear occurs, typically when the muscle is contracted, there is a bulge at the muscle-tendon junction where the muscle is still attached to the bone (Garrett 1990).
Muscle strain injury occurs in two ways, a stretch of the muscle to complete rupture or, more commonly, an incomplete injury in which there is not a complete separate of the tissue to the level of complete tear (Garrett 1990). A strain injury tends to result in bleeding; this bleeding may occur immediately post-injury, but there is a possibility of experiencing a delay prior to the detection of the subcutaneous ecchymosis of one or more days (Garrett 1990). This bleeding is not confined to the muscle proper; the bleeding also escapes through the perimysium and the fascia into the subcutaneous space (Garrett 1990). Computed tomography has confirmed that within the muscle tissue there is also an inflammatory or edematous response that occurs in response to muscle strain injury (Garrett et al. 1989). Ultrasonography of strain injury has demonstrated that bleeding and hematoma can collect between the muscle tissue and the surrounding fascial compartment as a result of the injury (Fornage et al. 1983).
McMaster, in 1993, completed one of the first studies describing injuries at the muscle-tendon junction (McMaster 1933). When there is a normally occurring stretch in the muscle-tendon junction, disruption of the tendon is not present (McMaster 1933). More likely than injuries to the muscle-tendon unit are injuries at the bone-tendon junction, the muscle-tendon junction, and the muscle substance tears (McMaster 1933). Immediate changes are demonstrated in the muscle-tendon junction following a controlled passive strain injury limited to the plastic region of the deformation curve; there is also a limited rupture of the most distal muscle fibers along with hemorrhage (Nikolaou et al. 1987). This is in agreement with previous studies examining the failure properties of the muscle-tendon units under passive extension, demonstrating that the muscle-tendon junction is the site of rupture and therefore the weakest point in the muscle-tendon unit (Nikolaou et al. 1986).
When failure occurs, the muscle fibers near the muscle-tendon junction fail as opposed to a clear separation of the tendon from the muscle fibers; in other words, a small amount of muscle tissue remains attached to the tendon (Garrett et al. 1988). When passive extension is utilized to create muscle strain injuries, those muscle-tendon systems that are deemed normal do not rupture within the tendon (Garrett et al. 1988). The passive extension leads to rupture at the tendon insertion, an avulsion of the muscle organ, a rupture in the muscle belly, a separation of the muscle-tendon junction, or a fracture of the bone (Garrett et al. 1988). During passive extension, the weakest point in the muscle-tendon unit has been identified as the muscle-tendon junction (Garrett et al. 1988). The distal end of the muscle-tendon junction, more specifically, has been identified as the weakest point in the muscle-tendon unit (Garrett et al. 1988). The muscle architecture plays an important role in the failure point, when the muscle is stretched (Garrett et al. 1988). A majority of muscles, regardless of type, fail at the muscle-tendon junction, more specifically at the distal muscle-tendon junction (Garrett et al. 1988). This does not hold true for the gastrocnemius, which is a multipennate muscle (Garrett et al. 1988). The multipennate architecture results in an arrangement of the muscle-tendon junction which is more complicated than other arrangements (Garrett et al. 1988). This architecture diminishes the ability to categorize the distal and proximal tears of the muscle-tendon junction, like can be done with more simplistic muscle architecture types (Garrett et al. 1988). For example, in experiments, 55.5% of gastrocnemius muscles stretched into passive extension have a clear failure point at the distal muscle-tendon junction (Garrett et al. 1988). The remaining percentage of failures occurs between the muscle-tendon junctions, separating the two heads of the gastrocnemius or within each gastrocnemius head along the deep tendon expansions (Garrett et al. 1988). Although these remaining failures cannot be categorized into distal or proximal muscle-tendon junction failures, they still follow a similar pattern of failure when compared to the other muscles tested and still occurred in the muscle-tendon junction (Garrett et al. 1988). Regardless of muscle architecture, when muscles are passively extended, no failures occur midbelly of the muscle fibers, within the tendon, or at the origin or insertion of the tendon to the bone (Garrett et al. 1988). Ruptures of the muscle-tendon junction do not cleanly separate the tendon from the muscle; during passive extension, when failure occurs, the tendon which is avulsed carries approximately 0.5 mm of the muscle fibers away with it (Garrett et al. 1988).
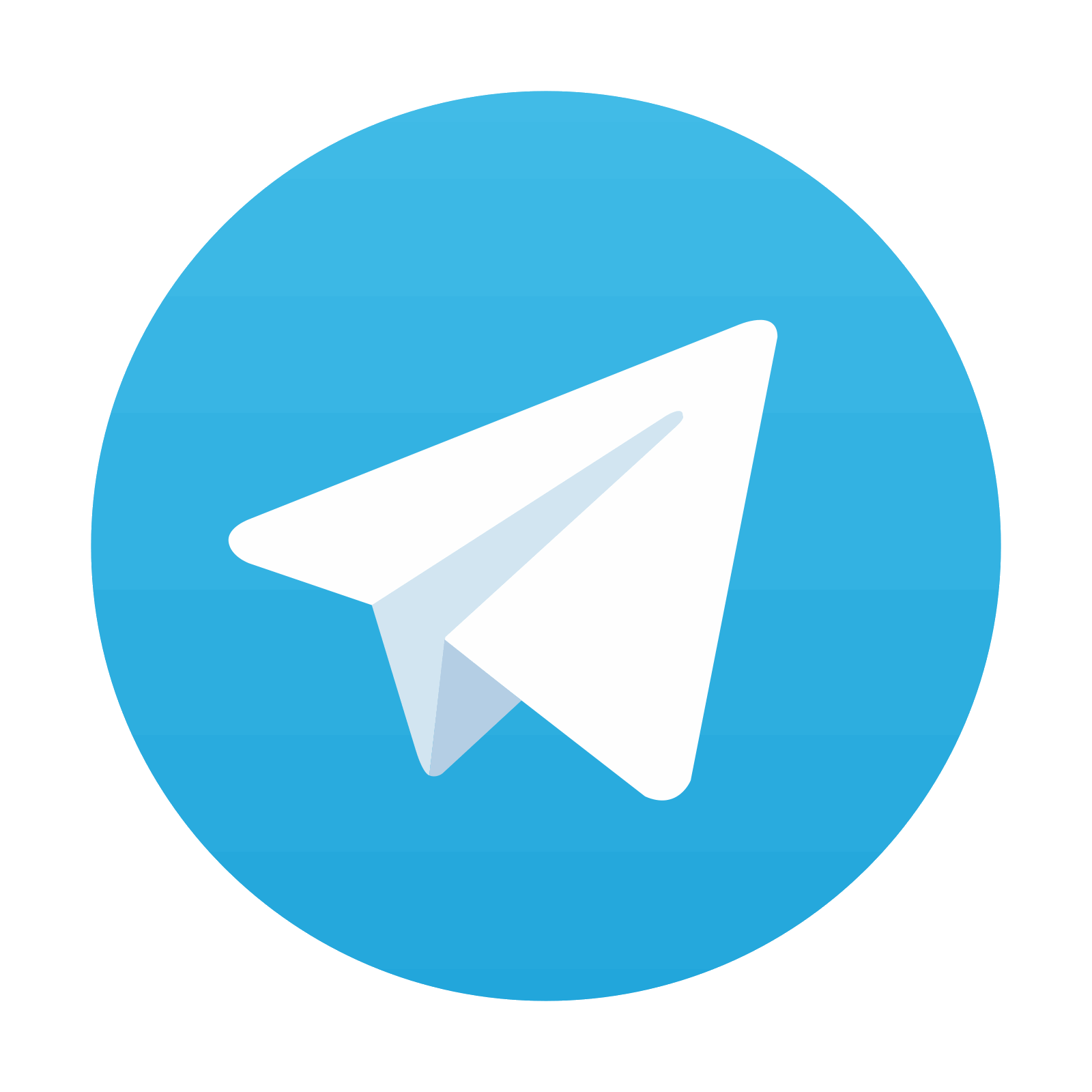
Stay updated, free articles. Join our Telegram channel
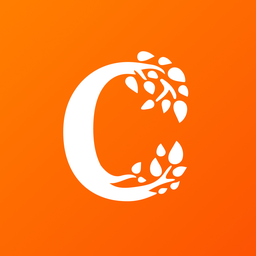
Full access? Get Clinical Tree
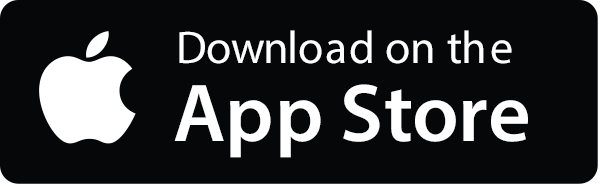
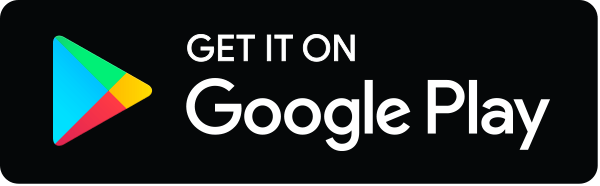