Materials Science and Biodegradable Scaffolds
Kenneth S. Ogueri, BS, MSc, PhD
Kevin Grassie, BS
Yusuf Khan, PhD
Cato T. Laurencin, MD, PhD, FAAOS
Dr. Ogueri or an immediate family member serves as a paid consultant to or is an employee of Pfizer. Dr. Khan or an immediate family member serves as a board member, owner, officer, or committee member of the Regenerative Engineering Society. Dr. Laurencin or an immediate family member has received royalties from CT Biotech and Globus Medical; is a member of a speakers’ bureau or has made paid presentations on behalf of Johnson & Johnson, Society for Science and the Public, Southern University of Science and Technology (SUSTech), Technion Israel Institute of Technology, University of California – San Diego, University of Oklahoma, University of Texas MD Anderson Cancer Cente, Vail Scientific Summit, and Western University of Health Sciences; serves as a paid consultant to or is an employee of Biorez, Bioventus, DePuy, Endo Pharmaceuticals, Johnson & Johnson, and Soft Tissue Regeneration; has stock or stock options held in Alkermes, BioBind, Healing Orthopaedic Technologies, HOT Bone, and Soft Tissue Regeneration, Inc.; has received research or institutional support from BioBind, Endo Pharmaceuticals, Healing Orthopaedic Technologies, and HOT Bone; and serves as a board member, owner, officer, or committee member of the National Academies, the National Academy of Inventors, and the Regenerative Engineering Society. Neither Kevin Grassie nor any immediate family member has received anything of value from or has stock or stock options held in a commercial company or institution related directly or indirectly to the subject of this chapter.
INTRODUCTION
Recent advances in materials science and engineering have spurred the design and development of sophisticated biomaterials with various properties and diverse functionalities. The advancements present leverage that ensures the fulfillment of the continuous demands and the understanding of the intricacies of regenerative engineering. Polymeric materials, including natural, synthetic, and composite, are poised to play a leading role in ensuring the effectiveness and success of matrix-based regenerative engineering. A holistic overview of the use of polymeric scaffolds in matrix-based strategies for tissue regeneration is provided, highlighting the underlying concepts and principles of several aspects of the regenerative process, such as osteoconductivity, osteoinductivity, and osseointegration. Moreover, the different fabrication methods typically used to ensure optimal scaffold geometry for enhanced biomaterial-tissue interactions and integration are discussed. The technology outlook and future direction of biodegradable scaffolds is outlined.
MATRIX-BASED REGENERATIVE ENGINEERING AND ITS COMPONENTS
The approach of regenerative engineering has seen consistent growth and advancements in recent decades. The progress is due to the quest for a new frontier in advancing the treatment of bone loss or failure. Regenerative engineering is an innovative and transdisciplinary approach that amalgamates and maximizes the principles of several areas of study such as advanced materials science and engineering, stem cell science, developmental biology, biophysics, and clinical translation for the regeneration of complex tissues and biologic systems1,2,3 (Figure 1). Essentially, the approach provides a platform that uses the expertise in these areas to design and develop the next-generation bone grafts. It encompasses bringing together stem cells, biocompatible and biomimetic scaffolds, and appropriate biochemical and mechanoelectrical cues to regenerate bone tissues. Scaffolding materials are used in matrix-based regenerative engineering as a structural template to arouse the body’s self-healing mechanism, leading to functional tissue development.4 The ultimate goal is to have a scaffold with appropriate instructive cues that can induce favorable cellular responses and regulate the formation of tissues. Considerable material science effort has been expended on the design of ideal biomaterials that can be used to fabricate biomimetic scaffolds with optimal topographic, biologic, and mechanical features required for effective tissue regeneration. A suitable scaffold possesses sufficient initial mechanical strength and presents a degradation rate that matches with the regeneration process while degrading into benign and resorbable degradation products. These scaffolding material attributes will be discussed in detail in the next section. Developmental biology provides a solid theoretic and practical basis in the processing of bone healing, unveiling the molecular and morphogenetic events fundamental to the formation and development of bone tissues. The functional insights gained from the field can be leveraged as a conceptual framework for directing and moderating early-stage tissue formation in regenerative engineering.
Developmental biology encompasses osteoinduction by using growth factors and small bioactive molecules to stimulate certain physiologic processes toward tissue formation. Bone morphogenetic proteins (BMPs)
are commonly used as potent osteoinductive factors because they facilitate stem cell mitogenesis that ultimately leads to cell division and differentiation toward osteoblasts. Stem cell technology is an essential component of regenerative engineering with remarkable traits of self-renewal and a tendency to differentiate into all types of cells. Stem cells used in regenerative engineering include mesenchymal stem cells (MSCs), embryonic stem cells, and induced pluripotent stem cells.4,5 Stem cell research and therapy have blossomed in recent years, and more stem cells are expected to be identified and developed, ensuring an unlimited source for a wide range of human cells needed for regenerative purposes. Biophysics has added another dimension to the regenerative toolbox because it aids in providing a good understanding of the cell mechanics and response to external stimuli such as stress within a bioengineered construct. The mechanical behavior of living cells relates to their functions because the cells produce and sustain mechanical stresses within their microenvironment as part of their normal physiology. This biomechanical stimulation plays a critical role in controlling and directing the morphogenetic actions of the cells, leading to the conversion of such forces into chemical signals that influence cellular behaviors. Several recent studies have illustrated that cells have the inherent ability to detect mechanical signals in their immediate environment. For example, the mechanical features of a cultured substrate can influence and manipulate the cellular fate because the cells tend to maneuver their ways to suit the mechanical profile of the substrate. Sometimes, they exhibit durotaxis, a phenomenon by which cells tend to drift toward specific mechanical conditions. As such, the control of the cell mechanics could be a valuable facet of regenerative engineering that can be exploited to optimize cell growth and organization.
are commonly used as potent osteoinductive factors because they facilitate stem cell mitogenesis that ultimately leads to cell division and differentiation toward osteoblasts. Stem cell technology is an essential component of regenerative engineering with remarkable traits of self-renewal and a tendency to differentiate into all types of cells. Stem cells used in regenerative engineering include mesenchymal stem cells (MSCs), embryonic stem cells, and induced pluripotent stem cells.4,5 Stem cell research and therapy have blossomed in recent years, and more stem cells are expected to be identified and developed, ensuring an unlimited source for a wide range of human cells needed for regenerative purposes. Biophysics has added another dimension to the regenerative toolbox because it aids in providing a good understanding of the cell mechanics and response to external stimuli such as stress within a bioengineered construct. The mechanical behavior of living cells relates to their functions because the cells produce and sustain mechanical stresses within their microenvironment as part of their normal physiology. This biomechanical stimulation plays a critical role in controlling and directing the morphogenetic actions of the cells, leading to the conversion of such forces into chemical signals that influence cellular behaviors. Several recent studies have illustrated that cells have the inherent ability to detect mechanical signals in their immediate environment. For example, the mechanical features of a cultured substrate can influence and manipulate the cellular fate because the cells tend to maneuver their ways to suit the mechanical profile of the substrate. Sometimes, they exhibit durotaxis, a phenomenon by which cells tend to drift toward specific mechanical conditions. As such, the control of the cell mechanics could be a valuable facet of regenerative engineering that can be exploited to optimize cell growth and organization.
As the last component, clinical translation entails the part of the regenerative tool that ensures the actual execution of the regenerative concepts in bone tissue repair and regeneration. It bridges the gap between science and clinical practice because it connects the findings of these different research areas to each other and ultimately to the patients. Clinical translation sets the pragmatic tone for investigating new techniques that can facilitate the effective translation of discovery into real applications. This also includes the navigation of the regulatory obstacles, ensuring safety and success of technology transfer. So, conglomerate expertise and knowledge from these various facets of regenerative engineering are leveraged to present a game-changing platform for positive clinical outcomes during the design process of bioengineered tissue constructs.
BIOMATERIAL DESIGN FOR BONE REGENERATIVE ENGINEERING
Bone regeneration is a complex and well-organized cascade of the physiologic process of bone formation that commonly takes place during routine healthy healing of fractures and bone remodeling. Certain medical conditions and disease states (such as infections, trauma, tumor resection, genetic defects, significant bone defects, and hormonal imbalance) can compromise the normal regenerative process in affected individuals. This necessitates substantial bone regeneration beyond the standard self-healing potential for skeletal reconstruction of significant bone defects. Several strategies such as allografts, autographs, and xenografts exist to augment the weakened regenerative process. Nonetheless, the effectiveness and availability of these treatment options are limited. An autograft is most widely used as a gold standard but has limited availability and potential donor morbidity. However, there are high chances of potential disease transmission for allografts and xenografts, and they could induce unfavorable immunogenic responses from the new host. To overcome the limitations of current treatment strategies, bone scaffolds based on biomaterials have been designed for bone regenerative engineering. Biomaterial-based scaffolds must meet certain critical criteria for them to be appropriate for bone regenerative engineering: (1) the material must be biodegradable, biocompatible, bifunctional, and completely benign; (2) the material must possess preprogrammed and predetermined degradation rates that correspond to the regeneration rates with adequate dimensional integrity; (3) the material should have suitable surface chemistry that could allow optimal cellular attachment and proliferation (osteoconductive); (4) the material should exhibit physicochemical features that mimic native bone tissues; (5) the material should be able to present three-dimensional (3D) porous microstructures with efficient interconnectivity and optimal pore size (150 to 350 µm) for accommodation of cell infiltration, tissue ingrowth, oxygen diffusion, vascularization, nutrient transport, and enhanced surface area for improved cell-material interactions; (6) the material should be able to encapsulate growth factors and small bioactive molecules such as BMPs and anti-inflammatory agents; and (7) the material should be capable of recruiting progenitor cells that promote the induction of bone growth (they are osteoinductive).1,6 It is important to note that the complexity and variations that exist for biologic tissues make it very difficult for a material to meet and satisfy all the requirements, as mentioned previously. For this reason, it can be categorically postulated that no one-size-fits-all material exists for bone scaffold design. As a result, it becomes valuable to have a design platform that allows the customization and modification of scaffolding materials’ attributes. For example, the pore size and distribution can be tailored and personalized to meet specific application requirements because living cells and biologic tissues differ in compositions, chemistries, and physical properties for different patients and body parts.6
Generally, tissues are composed of a cluster of cells embedded in extracellular matrix (ECM), and the ECM plays a vital role in the overall well-being of the cells. The main functions of ECM are to support cell growth, promote differentiation, and supply essential nutrients to the cells. Several studies have demonstrated these characteristics where ECM was used as a structural template and an avenue to supply essential nutrients and growth factors for optimal cell growth.6,7 Thus, effective regeneration cannot be achieved by just supplying cells to the site of injury because both ECM and cells are lost because of injury when it comes to large-size defects.1 So, it becomes necessary to devise an environment that architecturally looks similar to the ECM environment for the seeded cells. Biomaterials can be used as a substitute for ECM because they can be designed to exhibit physical attributes that mimic ECM proteins. Scaffold fabricated from biomaterial aims to mimic the ECM in a regenerating bone environment. Several biomaterials, including biopolymers, bioceramics, and composites, have shown great promise in regenerative engineering. These regenerative materials have unique properties that make them good candidates and appropriate for cell recruitment purposes. They have to be informative to cells and also provide structural support. Polymers are well known for their excellent tunability and mechanical properties, and ceramics have well-investigated effectiveness as bone scaffolds due to their similarity (in structure and chemical composition) with the main mineral phase of bone.
Mechanical competence is an essential requirement for regenerative materials as the biomaterial scaffolds must present mechanical attributes that correspond with the anatomic site they are meant to be implanted. Sometimes, pure materials may not be capable of providing all the needed mechanical attributes by themselves. However, the mechanical properties can be enhanced by designing composite materials that synergistically combine the desirable properties of two different materials. At least one of the components will provide biocompatibility, and the other will offer mechanical strength, making the composite suitable for tissue regeneration. Despite the versatile design tools and a continuum of materials at the orthopaedic surgeon’s disposal, no material developed over recent decades can boast of having an exact footprint of the native bones.
ROLES OF BIOMATERIALS IN BONE REGENERATIVE ENGINEERING
Osteoconduction
For a biomaterial to be suitable for matrix-based regenerative engineering, it must have a certain degree of osteoconductivity that allows the formation of new bones on
its surface.8 Specifically, an osteoconductive surface of a bone implant stimulates and supports cell attachments, migration, proliferation, differentiation, and deposition of ECM of osteoprogenitor cells within the defect area. These are critical early stages of new bone formation. The formation of thin carbonated hydroxyapatite on a material surface causes protein adsorption, and this is instrumental to the attachment of bone-forming cells and subsequent activities related to bone matrix deposition. The integration of the newly formed bone into the bone tissue microenvironment or the stable anchorage of an implant obtained by the direct bone-to-implant contact is instigated by osteoconductivity of material through the apposition of bone mineral. Hence, osteoconductivity is essential to achieving functional bone regeneration. The physicochemical properties of biomaterials have a controlling influence on their osteoconductivity during bone healing, and as such, they can be used to exert control on cellular behavior. The osteoconductivity-controlling features of biomaterials include chemical composition, surface chemistry, morphology, architectural geometry, etc. As mentioned previously, certain biomaterials such as bioceramics (hydroxyapatite and tricalcium phosphate) and bioglass depict enormous osteoconductivity due to their similarity to natural bone minerals. In addition, some natural polymers such as collagen could possess excellent osteoconductivity, attributable to their compositional and structural characteristics that foster mineral deposition and mineralization through binding of noncollagenous matrix proteins.1,6
its surface.8 Specifically, an osteoconductive surface of a bone implant stimulates and supports cell attachments, migration, proliferation, differentiation, and deposition of ECM of osteoprogenitor cells within the defect area. These are critical early stages of new bone formation. The formation of thin carbonated hydroxyapatite on a material surface causes protein adsorption, and this is instrumental to the attachment of bone-forming cells and subsequent activities related to bone matrix deposition. The integration of the newly formed bone into the bone tissue microenvironment or the stable anchorage of an implant obtained by the direct bone-to-implant contact is instigated by osteoconductivity of material through the apposition of bone mineral. Hence, osteoconductivity is essential to achieving functional bone regeneration. The physicochemical properties of biomaterials have a controlling influence on their osteoconductivity during bone healing, and as such, they can be used to exert control on cellular behavior. The osteoconductivity-controlling features of biomaterials include chemical composition, surface chemistry, morphology, architectural geometry, etc. As mentioned previously, certain biomaterials such as bioceramics (hydroxyapatite and tricalcium phosphate) and bioglass depict enormous osteoconductivity due to their similarity to natural bone minerals. In addition, some natural polymers such as collagen could possess excellent osteoconductivity, attributable to their compositional and structural characteristics that foster mineral deposition and mineralization through binding of noncollagenous matrix proteins.1,6
Conversely, biomaterials of low biocompatibility, such as copper and silver, could generally exhibit little to no osteoconduction. Meanwhile, it is a common practice to introduce osteoconductive attributes to materials (such as metals, ceramics, and synthetic polymers) that generally have poor osteoconductivity through several methods such as coating and composite. Several studies have reported the feasibility of improving the osteoconductivity of synthetic polymers by the surface treatment with calcium phosphate (CaP) or the integration of CaP as the second phase in a composite system.9
Osteoinduction
Osteoinductivity is the biomaterial property that entails the induction of osteogenesis, which is regularly seen in any bone-healing process.10 The osteoinduction process practically involves the mesenchymal and osteoprogenitor cell recruitment and their stimulation to more specialized bone-forming osteoblasts in an ectopic bone formation in vivo. Although the exact mechanism behind osteoinduction remains elusive, innovative biomaterials with osteoinductive potential have emerged. In recent years, cell and molecular biology advancements have dramatically improved understanding of the phenomena.6 Two general prerequisites facilitate the phenomenon: (1) the presence of a matrix/scaffold that constitutes 3D porous structures that could accommodate and foster cell filtration; and (2) the cell recruitment to the defect site for the elevation of osteogenesis.6,11 Osteogenic bone formation is activated through the local accumulation of growth and differentiation factors such as BMP families, fibroblast growth factors, and bone-associated ions (calcium and phosphate).9 The influence of osteoinductive biomaterials on bone regeneration occurs at multiple levels. At the tissue level, osteoinductive biomaterials serve as active facilitators for oxygen diffusion, nutrient transport, and waste exchange between the material and tissue. Vascularization of the materials, which enhances tissue growth, is also stimulated. At the cellular level, osteoinductive biomaterials tend to initiate the differentiation of stem cells/osteoprogenitor cells toward osteogenic lineage by forming a carbonated apatite layer. The release of calcium and phosphate ions could signal cell chemotaxis, which involves the migration and direction of multiple cell types at the implantation sites. At the molecular level, osteoinductive materials tend to accrue osteogenic proteins such as BMP-2 and BMP-7 because of their great affinity with the surrounding proteins (osteoinductive) within the tissue microenvironment. A sequence of cellular activities on the biomaterial surface could be coordinated and encouraged by the accumulation and supplementation of local growth factors.12 Moreover, the enrichment of calcium and phosphate ions could result in supersaturation in the void of implants, accelerating the mineralization in bone formation. To date, CaP-based bioceramics are the most commonly used osteoinductive biomaterials for tissue regeneration.
Another noteworthy and essential feature of osteoinductive biomaterials is their porous macrostructure. Essentially, porosity is necessary for osteoinductivity because bone induction has not been observed on a flat surface. Instead, bone formation has always transpired in the pores within implants, where there is supersaturation of calcium and phosphate ions. This concept was confirmed in a study by Barradas et al,10 in which they demonstrated a direct correlation between microstructure and osteoinductivity of biomaterials. Based on the study, changes in porosity and roughness of the implants induced different levels of bone formation. For instance, surface-treated titanium implants with microstructural porosity could cause enormous bone induction as opposed to the untreated titanium that showed no sign of bone formation.13 Thus, the importance of adequate osteoinductivity of a biomaterial for regenerative engineering cannot be overemphasized.
Vascularization
Vascularization is an essential aspect of bone regeneration as the formation of blood vessels is needed for tissues with a size greater than 200 µm (oxygen diffusion limit in
vivo). The development of the vascular system allows the integration of newly formed vessels with the host blood supply, presenting a driving force for functional bone formation. The newly formed vessels play a role in ensuring an adequate supply of nutrients such as glucose and oxygen to the surrounding cells and the removal of metabolic by-products, such as carbon dioxide, lactate, and urea. The vascular network also participates in the regeneration process by helping to recruit progenitor cells to the defect sites.14,15,16,17 When bone injury or trauma occurs, a local oxygen deficiency (local hypoxia microenvironment) causes the blood vessels to invade the bone defects.5,18 However, this spontaneous process occurs much slower than the tissue healing rates, resulting in severe hypoxia and ultimately failure of bone regeneration.9,19 Given the pressing need to establish a functional vascular network during bone regeneration, various biomaterials demonstrated the ability to improve several aspects of vessel network formation. These materials have been used to fabricate scaffolds for bone regenerative engineering.1,6 Bone scaffolds could serve as a provisional framework that mediates the progenitor cells and the pericyte migration, offering structural support for nascent capillary sprouts. The architecture of the scaffolds during fabrication can be fine-tuned to optimize their effect on tissue-engineered constructs.20 A 2013 study by Choi et al21 reported a significant improvement in vascular network formation using inverse opal scaffolds with tiny pores. Recent advancements in scaffold fabrication techniques have spurred the development of 3D printing, which is being used to design more perfusable engineered tissue constructs. 3D-printed perfusable vascular systems populated with endothelial cells have shown good maintenance of metabolic function of tissue during regeneration of primary hepatocytes. There is a direct interaction between the endothelial cells and the chemical components of the biomaterials, and as such, vascularization can be influenced by the chemical composition of the scaffolding materials. Although most of the biomaterials used as regenerative materials exhibit high biocompatibility with endothelial cells, some have a proangiogenic potential for tissue healing. For example, silicate bioceramic (akermanite) has been shown to induce angiogenesis successfully by releasing an optimal amount of silicon ion that stimulated human aortic endothelial cell proliferation and gene expression. Hydroxyapatite biomaterials are capable of regulating vascularization due to their high affinity to angiogenic cytokines such as vascular endothelial growth factor (VEGF), endothelial growth factor, and basic fibroblast growth factor. Inspired by the interaction between these biomaterials and bioactive molecules, there is the sequestration of endogenous growth factors at the defect sites and the ultimate improvement of bone formation via an enhanced vascular network development. Techniques such as the integration of growth factors (VEGF and basic fibroblast growth factor) with biomaterial scaffolds have been used to increase the vascularization of the tissue-engineered construct.7,20 The VEGF-encapsulated biomaterials intend to achieve a certain level of vascularization by promoting the proliferation of endothelial cells and the formation of a vascular network. Hence, the need to develop a vascular network may require that the biomaterial possess the ability to regulate and maintain VEGF release.
vivo). The development of the vascular system allows the integration of newly formed vessels with the host blood supply, presenting a driving force for functional bone formation. The newly formed vessels play a role in ensuring an adequate supply of nutrients such as glucose and oxygen to the surrounding cells and the removal of metabolic by-products, such as carbon dioxide, lactate, and urea. The vascular network also participates in the regeneration process by helping to recruit progenitor cells to the defect sites.14,15,16,17 When bone injury or trauma occurs, a local oxygen deficiency (local hypoxia microenvironment) causes the blood vessels to invade the bone defects.5,18 However, this spontaneous process occurs much slower than the tissue healing rates, resulting in severe hypoxia and ultimately failure of bone regeneration.9,19 Given the pressing need to establish a functional vascular network during bone regeneration, various biomaterials demonstrated the ability to improve several aspects of vessel network formation. These materials have been used to fabricate scaffolds for bone regenerative engineering.1,6 Bone scaffolds could serve as a provisional framework that mediates the progenitor cells and the pericyte migration, offering structural support for nascent capillary sprouts. The architecture of the scaffolds during fabrication can be fine-tuned to optimize their effect on tissue-engineered constructs.20 A 2013 study by Choi et al21 reported a significant improvement in vascular network formation using inverse opal scaffolds with tiny pores. Recent advancements in scaffold fabrication techniques have spurred the development of 3D printing, which is being used to design more perfusable engineered tissue constructs. 3D-printed perfusable vascular systems populated with endothelial cells have shown good maintenance of metabolic function of tissue during regeneration of primary hepatocytes. There is a direct interaction between the endothelial cells and the chemical components of the biomaterials, and as such, vascularization can be influenced by the chemical composition of the scaffolding materials. Although most of the biomaterials used as regenerative materials exhibit high biocompatibility with endothelial cells, some have a proangiogenic potential for tissue healing. For example, silicate bioceramic (akermanite) has been shown to induce angiogenesis successfully by releasing an optimal amount of silicon ion that stimulated human aortic endothelial cell proliferation and gene expression. Hydroxyapatite biomaterials are capable of regulating vascularization due to their high affinity to angiogenic cytokines such as vascular endothelial growth factor (VEGF), endothelial growth factor, and basic fibroblast growth factor. Inspired by the interaction between these biomaterials and bioactive molecules, there is the sequestration of endogenous growth factors at the defect sites and the ultimate improvement of bone formation via an enhanced vascular network development. Techniques such as the integration of growth factors (VEGF and basic fibroblast growth factor) with biomaterial scaffolds have been used to increase the vascularization of the tissue-engineered construct.7,20 The VEGF-encapsulated biomaterials intend to achieve a certain level of vascularization by promoting the proliferation of endothelial cells and the formation of a vascular network. Hence, the need to develop a vascular network may require that the biomaterial possess the ability to regulate and maintain VEGF release.
CELL-BIOMATERIAL INTERACTIONS
At the cellular level, the interaction of biomaterials and surrounding cells has a controlling or modifying influence on bone regenerative engineering because the nature and characteristics of biomaterials determine the regeneration outcome.11 Cell-biomaterials interactions occur in sequential manners that involve three different stages: adhesion, proliferation, and differentiation. As an integral aspect of the cell-material interactions, cell adhesion plays a central role of mediating and dictating the cellular behaviors on the surface of biomaterials. Integrins, heterodimeric and transmembrane receptors, are responsible for the adhesion between cells and substrate through the activation of a series of intracellular signaling pathways that transmit the character of the matrix to the cells.9 Integrins are composed of a diverse family of glycoproteins highly organized as heterodimers. These heterodimers can specifically bind onto the surface of a variety of matrix constituents. Integrin-mediated cell adhesion is a critical determinant of subsequent cell activities including cell morphology, motility, proliferation, and differentiation.20
In general, most of these interactions occur on the surface of the biomaterials, and hence the surface properties such as chemical composition, hydrophilicity, and topography of biomaterials have an enormous effect on cell fates.11,19 The chances of attaining optimal cell responses are maximized by directing the adsorption of proteins at the cell-biomaterial interface because the materials are immediately covered with protein layers from the environment once implanted.7,20 This mechanism has motivated the development of a series of surface modification and functionalization strategies that have been vastly extended to integrin-ECM molecule interactions. Numerous ECM macromolecules such as collagens, laminins, fibronectin, and vitronectin have been coated on various biomaterial surfaces to precisely control cellular performance on these surfaces. For example, bone substitutes based on collagen and its derivatives have been extensively used as implants to facilitate and improve material-cell interactions on implantation. The arginine-glycine-aspartic acid motif derived from fibronectin is the most widely investigated cell-binding peptide and has been used in several studies to enhance cell attachments. Arginine-glycine-aspartic acid motif has been blended with some bioinert systems, such as
poly(ethylene glycol) (PEG), to control material property for selective cell behavior studies.6
poly(ethylene glycol) (PEG), to control material property for selective cell behavior studies.6
Outside of protein-mediated interactions, other material surface characteristics can also have a remarkable effect on bone-forming capability by mediating cellular behaviors during bone regeneration. The importance of surface chemistry and chemical composition has been demonstrated in various orthopaedic devices and bone scaffolds surface treated with CaP.6 The surface energy of biomaterials can influence the capacity for new bone formation by affecting the response of the osteoblasts. Olivares-Navarrete et al19 found that osteoblast differentiation was improved on titanium surface with increasing surface energy. The topography of biomaterials has also been proven to affect bone formation. Lu et al22 pioneered the work that confirmed this topographic effect, showing enhanced osteoblast alignment on grooved titanium (without a compositional change) as opposed to titanium without groove. More recent studies have channeled efforts in microfabrication and nanofabrication methods that have the potential to create multiscale physical features that favor bone formation. Webster et al23,24 identified that nanoscale features (<100 nm) of biomaterials could be recognized by osteoblasts, leading to altered cellular responses and activities.
Furthermore, cell-material interactions depend on the mechanical properties of biomaterials because the cells react to mechanical stimuli such as the stiffness gradient of ECM via mechanotransduction.1 The mechanotransduction process occurs through mechanosensitive ion channels, the forced unfolding of proteins, and remodeling of focal adhesion sites. In particular, changes in the stiffness of the matrix could induce different cellular responses, which could serve as an important driving force for cellular behaviors.25,26,27 For example, MSCs seeded on a matrix with the stiffness of the brain differentiated into neural cells, whereas the same MSCs turned to osteogenic cells when they were brought in contact with a matrix whose stiffness is the same as the bone.11
Integration With Host Tissue
The integration of newly formed bone tissue with the native microenvironment is one of the requirements for functional bone regeneration.28 During the course of this process, biomaterials assume an essential role because they serve as a scaffold for cell infiltration and tissue deposition and provide an avenue for the release of inductive signals. These biochemical cues facilitate tissue connection with the surrounding host networks, including the vasculature and the nervous system.13,19,29 The initial step of tissue integration entails supporting cell adhesion on the surface of scaffolds fabricated by various biomaterials. These biomaterial-based scaffolds are designed to present porous structures that support adequate nutrient transport and oxygen diffusion, allowing cell migration and population within the scaffolds. In the next stage, infiltrated endothelial cells and pericytes reorganize and form capillaries, which are critical to sustaining the viability of newly formed tissues.11 Ideal biomaterials support the formation and stabilization of vascular networks with an appropriate combination of chemistry and microstructure.11 Meanwhile, osteoblasts may begin to deposit a large amount of tissue matrix such as collagen and minerals on the structure of the scaffolds. Finally, the remodeling process regulated by osteoblasts connects the newly formed ECM to the natural ECM. The remodeling process must match the degradation rate of the scaffold for optimal integration of the newly formed bone with the host bone tissue. Generally, biomaterials have a bearing influence on bone tissue integration because they serve as mediators and regulators in multiple stages of bone formation.
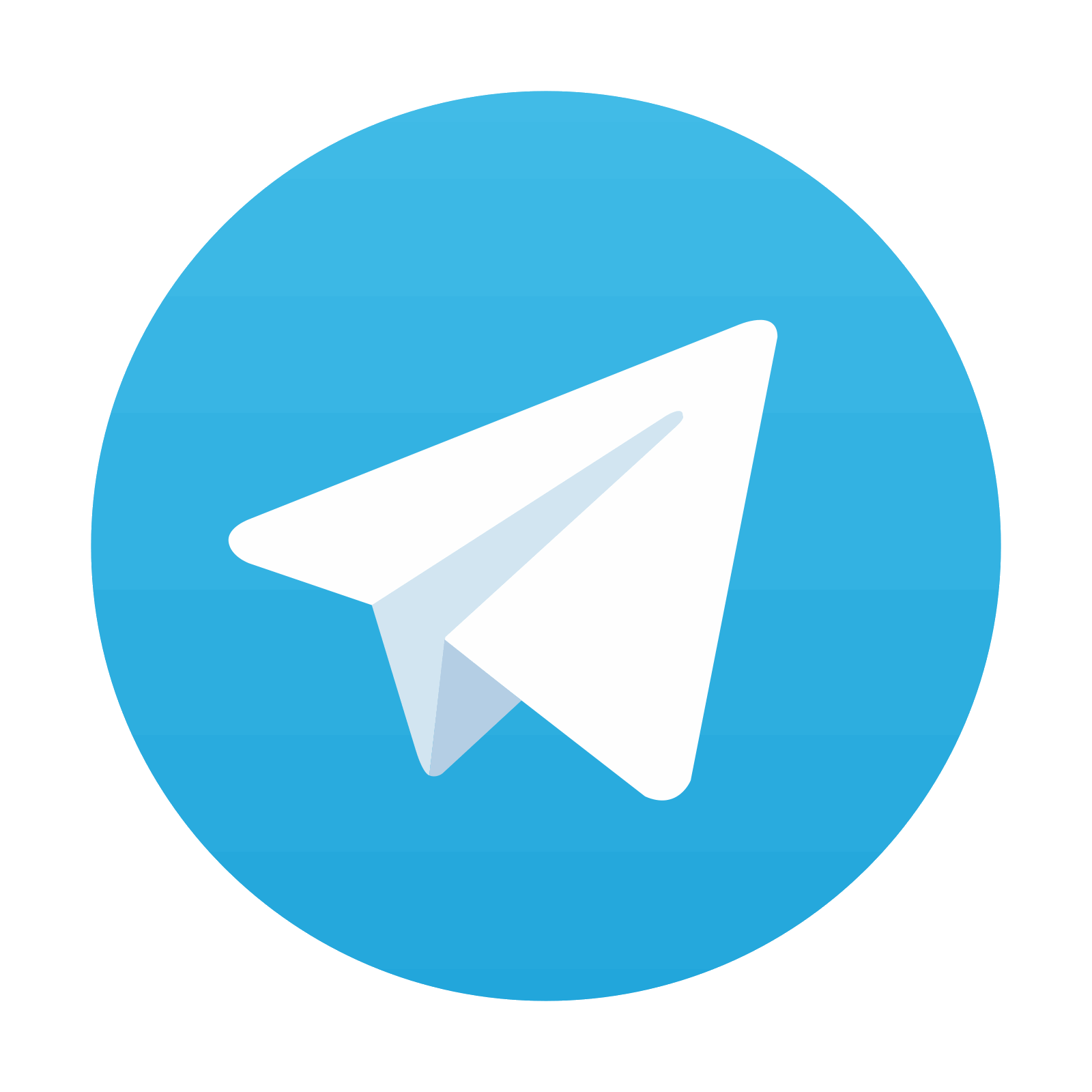
Stay updated, free articles. Join our Telegram channel
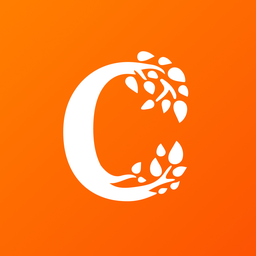
Full access? Get Clinical Tree
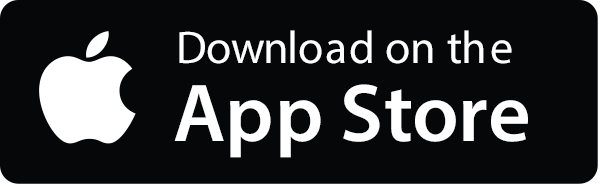
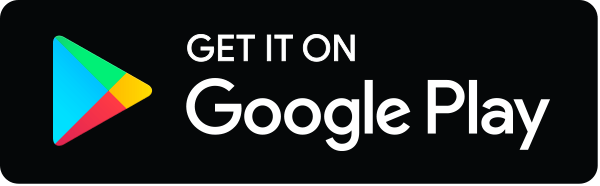