Fig. 6.1
Illustrations of (a) a normal glenoid, (b) a fractured anteroinferior glenoid (bony Bankart lesion), and (c) an “inverted pear” glenoid.

Fig. 6.2
Illustration showing the articular congruency of the glenohumeral joint. Note that only approximately 25 % of the humeral head articular cartilage makes contact with the glenoid throughout the entire arc of motion.

Fig. 6.3
Illustrations demonstrating (a) the effective glenoid arc and (b) the balance stability angle. The effective glenoid arc refers to the radius of curvature of the glenoid able to support joint reaction forces across the joint that would otherwise lead to humeral head translation. The balance stability angle is the maximum scapulohumeral angle at which humeral head translation can be prevented by the effective glenoid arc when an axial load is applied through the humerus.

Fig. 6.4
Axial cut-away view showing the structure of the glenoid, articular cartilage, and labrum.
Glenoid Version
Churchill et al. [12] reported that the normal glenoid is retroverted a mean of 1.2°. Their study reported a range between 9.5° of anteversion to 10.5° of retroversion in 344 human scapulae with a mean age of 25.6 years, indicating that a high degree of anatomic variability exists across the general population. Although it is unknown whether these shoulders were afflicted with recurrent instability, most evidence suggests that excessive glenoid version (anterior, posterior, superior, or inferior) or humeral torsion may be associated with decreased glenohumeral stability [12–17] and rotator cuff tears (Fig. 6.5) [18].


Fig. 6.5
(a) Increased glenoid retroversion can lead to recurrent instability due to absence of the effective glenoid arc. Severe anterior instability may cause tearing of the subscapularis tendon. (b) Increased humeral retrotorsion can lead to recurrent instability by overcoming the native balance stability angle of the glenohumeral joint.
Coracoacromial Arch
The coracoacromial arch is situated anterosuperiorly above the humeral head and is composed of the anterior acromion and the coracoid with the coracoacromial ligament spanning between these structures. This arch is known to prevent excessive anterosuperior migration of the humeral head (Fig. 6.6). However, contact of the humeral head with the undersurface of the acromion can be both a cause and effect of significant rotator cuff disease (see Chap. 4). In general, clinical instability as a result of superior humeral head migration in the absence of a large rotator cuff tear is an extremely rare entity.


Fig. 6.6
(a) Normal coracoacromial arch. (b) Surgical release of the coracoacromial ligament and removal of the anterior acromion can result in anterosuperior instability. This is extremely uncommon when the rotator cuff is intact.
Glenoid Labrum
The glenoid labrum is a triangular, fibrocartilaginous structure that adheres to the circumference of the glenoid rim (see Fig. 6.1). Its primary function is to provide an extension of the bony glenoid by increasing both its depth and surface area (Fig. 6.7)—factors that have been shown to contribute to approximately 10 % of glenohumeral stability [19, 20]. Recently, Park et al. [21] studied the effect of labral height on subjective outcomes in 40 patients who underwent arthroscopic repair of soft-tissue lesions of the anteroinferior glenoid (i.e., Bankart lesions). Patients with decreased labral height after repair demonstrated inferior clinical outcomes 1 year postoperatively (via Rowe scores) when compared to those with higher labral height. In addition to improving glenoid depth and contact surface area, the glenoid labrum also serves as an attachment site for the joint capsule and the glenohumeral ligaments. Approximately 40–60 % of the LHB substance originates from the superior labrum (the remaining percentage originates from the supraglenoid tubercle) (Fig. 6.8) [22].



Fig. 6.7
(a) Effective glenoid depth with an intact labrum. (b) Effective glenoid depth with a labral tear.

Fig. 6.8
Cadaveric photograph showing the origin of the LHB tendon from both the superior labrum and the supraglenoid tubercle.
Capsuloligamentous Structures
With the humerus in a neutral position (such as the “loose pack position” described in Chap. 2), stability is achieved through dynamic muscle contraction since the glenohumeral joint capsule and associated ligamentous structures are somewhat lax in this position [23]. However, these structures become variably taught with both active and passive shoulder motion which both maximizes articular surface contact and prevents abnormal humeral head translation (Fig. 6.9). The joint capsule itself, the coracohumeral ligament (CHL) and the superior, middle, and inferior glenohumeral ligaments (SGHL, MGHL, and IGHL, respectively) make up this important capsuloligamentous complex (Fig. 6.10).



Fig. 6.9
(a) Loose pack position. Note that in the scapular plane, the humerus and glenoid are in neutral alignment and the anterior and posterior capsule are in a normal resting position. (b) Increased tension of anterior structures occurs when the humerus is externally rotated. The posterior structures become taut when the humerus is internally rotated.

Fig. 6.10
Illustration showing the major labroligamentous structures surrounding the glenoid. The rotator cuff musculature is also shown.
The glenohumeral joint capsule is one of the primary static restraints involved in maintaining shoulder stability. It is primarily composed of collagen fiber bundles with varying degrees of thickness and fiber orientation [24, 25]. The CHL, SGHL, MGHL and IGHL make up distinct bands or areas of capsular thickening that are important for the maintenance of glenohumeral stability in any plane of shoulder motion. These structures become variably taught with both active and passive motion, thus maximizing articular surface contact and preventing abnormal humeral head translation. The shape and function of each individual ligament is determined by the position of the humerus in space.
The CHL has two distinct bands (anterior and posterior) that originate from the lateral base of the coracoid and travel between the supraspinatus and subscapularis tendons. The anterior band merges with the insertional fibers of the subscapularis tendon and the joint capsule near the lesser tuberosity whereas the posterior band inserts over the anterior aspect of the greater tuberosity. With the arm at the side, the anterior band primarily resists excessive external rotation and the posterior band primarily functions to prevent excessive internal rotation. Both the anterior and posterior bands of the CHL also provide resistance to inferior humeral head translation with the arm at the side and posterior humeral head translation when the arm is horizontally adducted [26–29].
The SGHL originates from the superior rim of the glenoid near the biceps-labral complex, travels parallel to the much larger CHL, and inserts on the lesser tuberosity, blending with the fibers of the subscapularis tendon. Its usual functions are similar to that of the CHL, preventing excessive external rotation [30] and inferior translation [31] when the arm is at the side and preventing posterior translation when the arm is horizontally adducted. However, its diameter, strength, and relative contribution to shoulder stability are highly variable across the population.
The anatomy of the MGHL is also highly variable. It can originate from the scapular neck, the anterosuperior glenoid rim or the supraglenoid tubercle with the biceps-labral complex. Similar to the CHL and the SGHL, the distal insertion of the MGHL blends with the fibers of the subscapularis tendon. The morphologic phenotype of the MGHL can also range in appearance from a round, cord-like ligament to a flat, sheet-like structure that blends with the IGHL inferiorly. Biomechanical ligament sectioning studies have shown that the MGHL primarily functions as a restraint to anterior translation when the humerus is between 0° and 45° of abduction and externally rotated [23]. In addition, the MGHL may also be important in limiting external rotation when the humerus is abducted greater than 60°.
The IGHL complex circumferentially attaches to the inferior aspect of the glenoid labrum anteriorly, inferiorly, and posteriorly and runs laterally to widely insert over an area extending between the lesser tuberosity anteriorly and the triceps tendon posteriorly. The IGHL is composed of thick anterior and posterior bands with an interposed “hammock-like” pouch that loosely cradles the inferior aspect of the humeral head (see Fig. 6.10). Its function is to resist both anterior and posterior humeral head translation when the humerus is abducted more than 60° [30]. Specifically, as the humerus is abducted and externally rotated, the anterior band of the IGHL complex along with the anteroinferior capsule becomes taut and prevents anterior humeral head translation. When the humerus is flexed, adducted, and internally rotated, the posterior band of the IGHL complex and the posterior capsule become taut and prevent posterior humeral head translation (see Fig. 6.9).
Rotator Interval
The rotator interval is a triangular space over the anterosuperior aspect of the joint capsule. The supraspinatus forms the superior border, the subscapularis forms the inferior border, and the coracoid process forms the medial base (Fig. 6.11). The CHL, the SGHL, the MGHL, the LHB tendon and the anterosuperior joint capsule all reside within this triangular space. Jost et al. [32] performed one of the more detailed cadaveric studies in which the rotator interval was described as being composed of several layers. However, the precise anatomy of the rotator interval is still under investigation and is beyond the scope of this chapter.


Fig. 6.11
Illustration highlighting the components of the rotator interval.
The exact function of the rotator interval is also the subject of numerous biomechanical studies [26, 32–39]. However, many of their reported results have been conflicting. Harryman et al. [36] performed one of the first comprehensive and descriptive studies that examined the function of the structures within the rotator interval. After dividing the capsule and ligamentous structures within the rotator interval in a series of 80 cadaveric shoulders, the investigators noted an increase in passive glenohumeral flexion, extension, external rotation, and adduction capacity. Medial–lateral imbrication of the same structures resulted in the opposite effect, thus decreasing these motions. The authors concluded that the rotator interval provided resistance against excessive motion while also functioning to limit posteroinferior glenohumeral translation. Nobuhara and Ikeda [39] also showed that tightening of the rotator interval decreased the propensity for humeral head translation in the posteroinferior direction. As a result of these studies, most surgeons believe that the rotator interval does provide some degree of stability, especially inferiorly when the humerus is externally rotated.
As mentioned in Chap. 5, the rotator interval also contributes to stability of the LHB tendon as it travels through the bicipital groove towards the superior labrum and supraglenoid tubercle. Specifically, the SGHL, CHL, and subscapularis tendon together form a structure known as the biceps reflection pulley which supports the tendon as it enters the glenohumeral joint (Fig. 6.12) [2, 26, 27, 40].




Fig. 6.12
(a) Illustration showing the structure of the bicipital sheath and biceps reflection pulley (BRP) as the LHB tendon travels into the glenohumeral joint. (b) Arthroscopic image showing anteromedial (AM) and posterolateral (PL) BRPs. (Part B from Elser F, Braun S, Dewing CB, Giphart JE, Millett PJ. Anatomy, function, injuries, and treatment of the long head of the biceps brachii tendon. Arthroscopy. 2011;27(4):581–92; with permission).
Negative Intra-Articular Pressure
Within the closed joint, a negative intra-articular pressure is produced by either a “piston-in-valve” mechanism, an adhesion-cohesion effect of the viscous synovial fluid, or both, which exist to provide some degree of stability to the glenohumeral joint [41]. Any perforation of the joint capsule can “vent” the joint, eliminating this nascent pressure gradient (Table 6.1) [41–43]. However, although this mechanism may provide some joint stability, capsular venting alone is not an apparent cause of clinical instability.
Table 6.1
Mean intra-articular pressures with the neutral and abduction/axial traction positionsa
Neutral position (mm Hg) | Abduction/axial traction (mm Hg) | |
---|---|---|
Cadaveric shoulders (n = 18) | −34 | −111 |
Stable shoulders (n = 15) | −32 | −133 |
Unstable shoulders (n = 17) | 0 | −2 |
6.2.1.2 Dynamic Constraints
Rotator Cuff
The rotator cuff contributes to glenohumeral stability through several different mechanisms. First, contraction of the rotator cuff muscles serves to compress the humeral head within the glenoid concavity, thus maximizing contact between the articular surfaces during active motion (the “concavity compression” mechanism is discussed below). Second, the physical presence of the rotator cuff musculature prevents humeral head migration. Specifically, the supraspinatus (along with the coracoacromial arch) helps prevent superior translation, the infraspinatus and teres minor resist posterior translation and the subscapularis helps to stabilize the joint anteriorly. Third, the rotator cuff forms direct attachments with the joint capsule and contributes to stability by increasing capsular tension during active motion. Finally, the glenohumeral joint capsule has proprioceptors that are activated by capsular stretching [44]. Afferent nerve impulses travel through the dorsal root ganglia and return via efferent fibers to produce contraction of the rotator cuff and deltoid muscles which effectively counteracts the initial stimulus (i.e., capsular stretching).
Periscapular Musculature
The periscapular muscles, including the trapezius, rhomboids, levator scapulae, serratus anterior, latissimus dorsi, and pectoralis minor, function in synchrony to optimize the position of the scapula during rotation, elevation, and horizontal adduction of the humerus, thus maintaining the humeral head in a centered position within the glenoid fossa in any motion plane (Fig. 6.13). See Chaps. 2, 3 and 9 for more information regarding basic scapulohumeral kinematics and related physical examination techniques.


Fig. 6.13
Illustration highlighting the anatomy of the periscapular musculature.
Concavity Compression
Contraction of the rotator cuff and deltoid muscles compresses the humeral head against the glenoid fossa during active motion (also known as “concavity compression”; Fig. 6.14). As discussed in Chap. 4, the rotator cuff and deltoid muscles produce parallel force vectors that act against the glenoid surface. Simultaneous contraction of parallel muscles on opposite sides of the joint (e.g., the subscapularis anteriorly and the infraspinatus posteriorly) compresses the humeral head into the glenoid while contraction of muscles on the same side of the joint (e.g., the supraspinatus and the deltoid superiorly) produces humeral head rotation (e.g., abduction). In addition, the relative strength of contraction of each muscle determines the plane of elevation or rotation. For example, if the concentric contraction strength of the subscapularis was 1.0 units and the eccentric contraction strength of the infraspinatus was 0.5 units, the net rotational moment would favor subscapularis and, thus, internal rotation with simultaneous glenohumeral compression would result. This dynamic mechanism favors both motion and stability and can be applied to other muscles and joints throughout the body.


Fig. 6.14
Illustration highlighting the important force couples that help maintain concavity compression and overall glenohumeral stability. (a) The combined actions of the deltoid muscle (D) and the rotator cuff (C) make up the transverse plane force couple and pull the humeral head medially towards the glenoid fossa. (b) The combined actions of the subscapularis (S) and the infraspinatus (I) make up the axial plane force couple and also work to drive the humeral head medially towards the glenoid fossa.
6.2.2 Anatomic Variations
Many of the structures described above have several anatomic variations that are important to recognize from a management perspective (Fig. 6.15). However, because these variations are not discernible by physical examination, an in-depth discussion of each variation would not be helpful in the context of this chapter. Rather, we have provided a summary of this information with their corresponding references in Table 6.2 in an effort to direct the reader towards the most relevant published evidence related to these anatomic variations. In addition, we recommend reviewing the article published by Tischer et al. [53] which provides a detailed discussion of the relevance of each anatomic variation as they relate to the arthroscopic management of instability.


Fig. 6.15
Illustrations showing the most common glenolabral anatomic variations. The sublabral recess, sublabral foramen, and Buford complex are shown.
Table 6.2
Anatomic variations of the glenoid and glenoid labrum
Structure | Anatomic variation | Prevalence | References |
---|---|---|---|
Glenoid | Teardrop glenoid with notch | 59 % | Anetzberger and Putz [5] |
Teardrop glenoid without notch | 29 % | Anetzberger and Putz [5] | |
Oval glenoid | 12 % | Anetzberger and Putz [5] | |
Glenoid labrum | Any anterosuperior variation | 13.4–25 % | |
Sublabral recess | Highly variable | ||
Sublabral foramen | 12–18.5 % | ||
Buford complex | 1–6.5 % |
6.3 Classification of Instability
Instability is typically described according to severity (microinstability, subluxation, or dislocation), direction (anterior, posterior, inferior, or multidirectional), and chronicity (acute, chronic, or acute on chronic). In other cases, instability can also be described as being voluntary or involuntary [54] and hereditary or acquired [55]. Although several classification systems have been proposed, none of these have been comprehensive nor have they been proven to adequately facilitate communication between physicians, guide treatment decisions or predict outcomes. In 1989, Thomas and Matsen [56] categorically divided those with instability into two distinct groups according to the distinctive characteristics of their condition. In one group, the acronym TUBS was used to describe individuals with Traumatic, Unilateral instability with a Bankart lesion that generally requires Surgical repair. The other group was described using the acronym AMBRI which included patients with Atraumatic, Multidirectional instability which was typically Bilateral, Responded to physical therapy and sometimes required Inferior capsular plication to prevent recurrence. In this group, many individuals are afflicted with underlying multiligamentous laxity who gradually develop instability as they age. However, although this classification system has some academic merit, its usefulness in clinical practice is very limited since most patients present with pathologic traits that overlap between the TUBS and AMBRI groups. For example, many patients with generalized hyperlaxity present with uni- or bi-directional instability [57] whereas up to one-fourth of patients with traumatic instability display evidence of contralateral involvement and increased capsular elastin content which suggests the possibility of familial inheritance [55]. This overlap indicates that the term “instability” most likely encompasses a continuous spectrum of pathologic features where the TUBS and AMBRI groups represent the terminal ends.
As a result, other classification systems were proposed to account for this continuum of pathologies associated with glenohumeral instability. Particularly noteworthy is the Stanmore classification developed by Lewis et al. [58] in 2004 in which the three points of a triangle represent the polar pathologic characteristics associated with instability (Fig. 6.16). Type I represented traumatic instability, type II represented atraumatic instability, and type III represented neurologic dysfunction and muscle patterning (i.e., voluntary instability). The line between types I and II corresponded with the spectrum of disease between traumatic (TUBS) and atraumatic (AMBRI) etiologies. The line connecting types II and III corresponded with the spectrum of disease in those with atraumatic instability and neurologic dysfunction. As one moves towards the apex of the triangle (i.e., towards the polar type I pathology), the involved pathology increasingly resembles the clinical picture of traumatic instability. The opposite is true when one moves towards the base of the triangle. While this system adequately represents the variability in clinical presentation, it fails to guide the clinician towards a specific treatment option for individual clinical scenarios.
In 2008, Kuhn et al. [59] systematically reviewed the literature to determine the frequency of various criteria that have been used to classify glenohumeral instability. The authors observed that four of these criteria were used in more than 50 % of the proposed classification systems. They also noted that a survey conducted by the American Shoulder and Elbow Surgeons (ASES) echoed these same results. As a result, a classification system that accounts for Frequency, Etiology, Direction and Severity of instability (FEDS system) was developed since these features were found to be the most important factors involved in treatment decision-making. Subsequent analysis revealed that the FEDS classification had high inter- and intra-rater agreement [60]. However, the major drawback of the FEDS system is its inability to categorize all patients who present with instability. Therefore, further studies that correlate this classification system with treatment outcomes are needed. With this information, clinical results could be predicted at the time of initial clinical evaluation.
Due to the lack of a single validated classification system for glenohumeral instability, the clinician must utilize the concepts derived from several different classification schemes and published literature to make individual treatment decisions based on the etiology and underlying pathologic lesions associated with the injury.
6.4 Pathoanatomic Features of Traumatic Instability
6.4.1 Soft-Tissue Defects
6.4.1.1 Capsular Distention
As mentioned above, the IGHL complex is the most important static restraint that prevents abnormal anterior–posterior humeral head translations. In the normal shoulder, anterior (or posterior) dislocation occurs when a force is applied to the humeral head that exceeds the peak load required to displace the humeral head from the glenoid fossa, to stretch and deform the anterior (or posterior) band of the IGHL complex and, in most cases of acute dislocation, detachment of the glenoid labrum (i.e., Bankart Lesions—discussed below). Once the microstructure of the ligament has been damaged via plastic deformation, a return to its previous shape and function is unlikely in most cases [61]. This is particularly true for the mid-substance of the ligament where strain to failure has been found to be less than that of the other portions of the ligament substance (e.g., the bony insertion sites) [62]. Bigliani et al. [62] noted that failure of the anterior band occurred either at its glenoid insertion (40 %), in its mid-substance (35 %) or at its humeral insertion (25 %). However, significant elongation of the ligament occurred regardless of the mode of failure in this study. After reduction of the joint, the anterior (or posterior) band of the IGHL complex remains irrecoverably elongated and patulous, thus substantially increasing the risk for future dislocations (Fig. 6.17). Rowe et al. [63] found that up to 28 % of patients with recurrent instability had some degree of capsular redundancy. Further-more, recurrent episodes of instability increase the severity of capsular distention which can lead to significant disability through a variety of other mechanisms [13, 64].


Fig. 6.17
Magnetic resonance arthrogram (MRA) of the right shoulder following an acute posterior glenohumeral dislocation. Note the significant distention of the posterior capsule (arrow).
6.4.1.2 Bankart Lesions
Detachment of the anteroinferior glenoid labrum (also known as a Bankart lesion) is thought to occur in up to 90 % of cases of traumatic anterior instability and has traditionally been referred to as the “essential lesion” of traumatic shoulder dislocation (Figs. 6.18 and 6.19b). When the soft-tissue defect is associated with periosteal stripping of the glenoid neck without medial displacement of the labral tissue, it is typically referred to as a “Perthes lesion” (Fig. 6.19c) [65]. Despite its near-universal presence in cases of traumatic instability [66, 67], soft-tissue Bankart lesions alone are not a frequent cause recurrent instability. Rather, the underlying cause is most often multifactorial with particular focus on redundancy and plastic deformation of the IGHL complex [68].



Fig. 6.18
Illustration depicting an anteroinferior labral tear (Bankart lesion).

Fig. 6.19
Axial cut-away view showing (a) a normal glenoid labrum, (b) a Bankart lesion, (c) a Perthes lesion, and (d) an ALPSA lesion.
6.4.1.3 ALPSA Lesions
The anterior labral periosteal sleeve avulsion (ALPSA) lesion is an entity similar to that of the Bankart lesion; however, in this case, the periosteum along the anterior glenoid neck elevates from the underlying bone in a “sleeve-like” pattern along with the IGHL-labrum complex which typically appears in a medialized position (Fig. 6.19d) [69].
6.4.1.4 SLAP Tears
Superior labral anterior to posterior (SLAP) tears (discussed in Chap. 5) are more common in overhead athletes probably as a result of the peel-back mechanism as described by Burkhart and Morgan [70] (Fig. 6.20). The deceleration phase of the throwing motion may also produce extraphysiologic eccentric loads on the biceps anchor that can result in tearing or rupture. Complete tears of the biceps anchor increased superior–inferior and anterior–posterior humeral head translation in a cadaveric study [71]. However, more recent evidence suggests that posterosuperior migration of the humeral head in overhead athletes as a result of posterior capsular contracture may produce a greater degree of anterior translation that can easily be perceived as clinical laxity (i.e., “pseudolaxity”). This perceived laxity is more likely to be the result of posterior capsule contracture rather than the presence of a SLAP lesion in these patients; however, it should be noted that SLAP tears that extend into the MGHL can also produce increased anterior humeral head translation. Chapter 5 provides further details regarding SLAP tears.


Fig. 6.20
Illustration showing the peel-back mechanism described by Burkhart and Morgan [70]. Increasing degrees of external rotation increases the torsional strain across the biceps anchor which can lead to SLAP tears.
6.4.1.5 HAGL Lesions
The humeral avulsion of the glenohumeral ligament (HAGL) lesion occurs when the insertion of the IGHL complex avulses or otherwise separates from the humeral neck (Fig. 6.21a). Although its incidence is relatively low, this injury most commonly occurs after a first-time anterior shoulder dislocation. When combined with a Bankart lesion, the anterior band of the IGHL complex is referred to as a “floating segment.” The same combination of injuries can also occur posteriorly thus involving the posterior band of the IGHL complex (Fig. 6.21b) [72, 73].


Fig. 6.21
(a) Axial image of HAGL lesion. (b) Axial MRI demonstrating a floating posterior HAGL lesion. (From Martetschläger et al. [72]; with permission).
6.4.1.6 Rotator Interval Lesions
Due to the significant anatomic variability inherent to the rotator interval, it is sometimes difficult to determine whether a physical finding is normal or abnormal. However, in our experience, laxity of the rotator interval can be detected on physical examination by inducing a sulcus sign of >2 cm when the humerus is externally rotated (discussed below).
6.4.2 Osseous Defects
6.4.2.1 Bony Bankart Lesions
Anterior shoulder dislocations can also create fractures of the anteroinferior glenoid rim (i.e., bony Bankart lesions; Fig. 6.22). These fractures can range in morphology and size depending on the direction of load transmission. Loss of bone from the anterior glenoid from any cause decreases glenoid concavity and increases the potential for recurrent dislocations. In general, as the size of the lesion increases, glenohumeral stability decreases [74]. Several biomechanical studies have shown that defects measuring more than one half of the glenoid length decrease joint stability by up to 30 % [75, 76]. Others have shown that soft-tissue Bankart repair is not adequate for defects involving at least 20–25 % of the inferior glenoid diameter [7]. Although there are numerous methods for measuring anteroinferior glenoid bone loss, discussion of their significance is beyond the scope of this chapter.


Fig. 6.22
(a) Illustration of an anteroinferior glenoid fracture (bony Bankart lesion). (b) Velpeau axillary radiograph of a right shoulder showing a fracture of the anterior glenoid.
6.4.2.2 Attritional Glenoid Bone Loss
Erosion of the anteroinferior glenoid rim as a result of repeated dislocations is another cause for glenoid bone loss (Fig. 6.23). These patients must rely on soft-tissue constraints to maintain anterior stability; however, these restraints are insufficient due to the capsuloligamentous stretching from previous anterior dislocations. Although these patients present similarly to those with other causes of instability, there are many fewer treatment options. For example, there is often no bony fragment that can be used for surgical fixation and, in many cases, soft-tissue repair would not be adequate to prevent recurrent instability [63]. Bony reconstruction of the anterior glenoid is typically indicated which may involve iliac crest bone grafting, the Latarjet procedure, or distal tibial osteochondral allograft (Figs. 6.24 and 6.25).




Fig. 6.23
(a) Axillary radiograph of a right shoulder demonstrating attritional bone loss involving the anterior glenoid. Note that the humeral head appears to be resting anterior to the axis of glenoid. (b) Axillary radiograph of a left shoulder demonstrating attritional bone loss involving the anterior glenoid. The humeral head appears to be positioned anterior to the glenoid axis.

Fig. 6.24
Illustration depicting the Latarjet procedure for the treatment of recurrent anterior instability in the setting of glenoid bone loss.

Fig. 6.25
Other bony reconstruction options for the treatment of glenoid bone loss include iliac crest bone grafting and distal tibial osteochondral allograft. (a) Surgical photograph demonstrating fixation of a bone graft to the anterior glenoid in a patient with recurrent anterior instability. (b) Example of an iliac crest bone graft. (c) Example of a distal tibial osteochondral allograft.
6.4.2.3 Hill–Sachs Lesions
The Hill–Sachs lesion is characterized by an impression fracture of the posterosuperior aspect of the humeral head. These fractures can occur as a result of anterior dislocation when the soft bone of the posterosuperior humeral head impacts the much harder bone of the anteroinferior glenoid rim. Although most lesions are small and generally do not affect glenohumeral stability, other larger lesions can cause recurrent dislocations especially in positions of 90° of abduction and 90° of external rotation (i.e., the 90/90 position) where the humeral head defect can “engage” with the glenoid rim (Fig. 6.26) [7, 77–79].


Fig. 6.26
(a) Hill–Sachs lesions can engage with the anterior glenoid when the humerus is externally rotated. Bone loss or fracture of the anterior glenoid can exacerbate the problem. Engagement of the Hill–Sachs lesion with the anterior glenoid can deepen the humeral head defect. (b) Axial computed tomography (CT) scan showing an engaging Hill–Sachs lesion in a patient with debilitating instability.
6.4.2.4 Glenoid Version
Glenoid version, especially retroversion, has been cited as an uncommon, but potential contributory factor involved in recurrent shoulder instability due to the absence of an effective glenoid arc (see Fig. 6.5a) [12, 80]. Due to conflicting data suggesting a possible link between mild glenoid version and recurrent instability, this entity is generally considered a diagnosis of exclusion after all other causes of recurrent instability have been ruled out [14, 81, 82]. On the other hand, more severe cases of glenoid version can result in debilitating instability (Fig. 6.27); most of these cases involve significant retroversion that lead to posterior instability.


Fig. 6.27
Axial computed tomography (CT) scan demonstrating severe glenoid retroversion. This patient presented with recurrent posterior instability.
6.5 Pathoanatomic Features of Atraumatic Instability
It is often difficult for clinicians to define which patients are afflicted with atraumatic instability because the effects of activities of daily living and/or sporting activities may lead to undetectable glenohumeral joint damage. The cumulative effects of this damage may lead to unilateral or bilateral instability without an apparent cause. However, some degree of genetic predisposition is implied when patients present with atraumatic bilateral shoulder instability [83]. For example, multidirectional instability (MDI) is defined as atraumatic anterior or posterior instability with a component of increased inferior translation. Studies have demonstrated increased elastin content in both the skin and capsular tissue of many patients with MDI [84] in addition to increased capsular volume [57, 85, 86] and, in some cases, laxity of the rotator interval [87]. These findings suggest that undiagnosed Ehlers–Danlos syndrome or multiligamentous laxity may be a substantial contributing factor involved in the development of instability in many of these patients. It should be also noted that traumatic and atraumatic instability can occur simultaneously in the same patient and thus should not be considered entirely independent from one another. For example, a patient diagnosed with MDI can also present to the clinic after a traumatic dislocation, potentially resulting in any of the pathologic lesions associated with traumatic instability (i.e., bony Bankart lesion, Hill–Sachs lesion, HAGL lesion, etc.).
6.6 Instability or Increased Laxity?
The semantic relationship between laxity and instability should be recognized and understood by all clinicians who evaluate patients with various shoulder pathologies. The term “laxity” refers to the normal physiologic motion allowed as a result of the position and tension of the ligaments that maintains stability of a joint [88–90]. Without this physiologic laxity, joint motion would not be possible. Because the shoulder requires a large range of motion, its physiologic laxity has a greater magnitude than the other joints within the body. Therefore, laxity testing in the shoulder requires that the clinician understands the difference between “normal” and “abnormal” joint motion as they relate to the entire clinical picture. Along the same lines, the clinician should also understand that increased joint laxity does not necessarily equate pathologic instability, even if this finding occurs unilaterally. As mentioned above, these conditions lie along a spectrum of disease that is most frequently and conveniently labeled as “instability.”
6.7 Quantifying Humeral Head Translation
Currently, there are three basic methods by which humeral head motion is quantified: (1) translation in millimeters, (2) translation as a percentage of the humeral head diameter, and (3) the sensations felt when the humeral head is translated. A fourth modality includes the use of instrumentation or imaging; however, these methods are currently under development.
6.7.1 Humeral Head Translation in Millimeters
There are four grades of anterior and/or posterior translation of the humeral head [91].
Grade 0 = minimal or no translation
Grade 1 = <10 mm of translation
Grade 2 = 10–20 mm of translation
Grade 3 = >20 mm of translation or subluxation
A similar system exists for the measurement of inferior translation [92–95]. The primary limitation of this method of measurement is its subjectivity—that is, each measurement is an approximation made by the examiner and extensive practice is needed before one becomes proficient and accurate. As of this writing, these methods of measurement have not been biomechanically or clinically validated; however, they are widely used in the setting of a busy clinical practice due to their convenience and, when performed by the most experienced clinicians, sufficient accuracy.
6.7.2 Humeral Head Translation as a Percentage of Humeral Head Diameter
Measurement of humeral head translation can also be estimated using the humeral head diameter as described by Cofield and Irving [96]. Specifically, the amount of translation as a percentage of the humeral head diameter is used. This method accounts for the size of the individual being tested and may theoretically provide a more accurate estimate of glenohumeral translation. However, several studies have provided conflicting results regarding the amount of translation that should be considered abnormal. Reported estimates for normal anterior and posterior translations have ranged from 0 to 50 % and from 26 to 50 %, respectively [79, 89, 93, 97–99]. In addition, humeral head diameters vary widely across the population and its estimation may be difficult without some sort of radiographic measurement. This method has not been formally validated for the measurement of humeral head translation and, in at least one case, has been reported as invalid [89].
6.7.3 Tactile Sensation of Humeral Head Translation
Another way to quantify humeral head translation is to report what is felt by the examiner when the humeral head is translated anteriorly or posteriorly. The primary advantage of the classification scheme is that the measurement does not rely upon absolute numbers to define certain pathologies. There are four grades of translation according to Hawkins and Bokor [100]:
Grade 0: Normal physiologic motion
Grade 1: Translation to the glenoid rim
Grade 2: Translation over the glenoid rim
Grade 3: Humeral head remains out of joint after examiner removes hands (i.e., “lock out”)
Levy et al. [94] investigated the reliability and accuracy of the original Hawkins system to detect humeral head translations in a series of 43 athletes. Two fellows in sports medicine, a senior orthopedic resident or an attending physician in orthopedic surgery performed all of the physical examinations. These researchers found an overall inter-rater reliability of less than 50 %. However, they found that the inter-rater agreement increased to a mean of 73 % when grades 0 and 1 were considered together. The intra-rater agreement also increased from 46 to 73 % when grades 0 and 1 were consolidated. Using this modified Hawkins scale, McFarland et al. [101] demonstrated an intra-rater reliability of 100 % and 86 % for anterior and posterior humeral head translations, respectively. As a result of these studies, the original Hawkins system was modified due to the difficulty in distinguishing between patients with grade 0 and grade 1 translations. Currently, grade 1 represents humeral head translation “not over the rim,” grade 2 represents “over the rim,” and grade 3 represents “lock out” (Fig. 6.28). However, the clinical significance of the modified Hawkins system is still heavily debated. For example, using the anterior and posterior drawer tests (described below), several studies have demonstrated grade 2 laxity in physiologically normal shoulders without clinical instability [102–104]. In addition, these studies also found that glenohumeral joint laxity may be increased in the non-dominant shoulder, suggesting that asymmetric findings with laxity testing is most likely normal in the majority of cases. Additional research is necessary to determine the applicability of the modified Hawkins classification as it relates to the diagnosis of shoulder instability.


Fig. 6.28
Illustration of the modified Hawkins classification of glenohumeral translation (grades 1, 2 and 3) [100].
6.7.4 Objective Instrumentation
In the shoulder, humeral head translation in any direction is primarily measured by tactile sensation and requires a great deal of practice and experience. When a clinician becomes proficient, abnormal joint motions of <1 mm can reliably be detected, especially when the patient is under general anesthesia. Although this practice has some element of subjectivity, it is widely accepted since there are currently no validated devices that can accurately and reproducibly detect small amounts of joint motion. These types of instruments for the shoulder are currently in development and use a similar design to that of the KT-1000 [105–107] a device used to measure tibial translation relative to the femur. However, measuring humeral head translation using these new instruments is limited due to the effects of soft-tissue compliance and patient apprehension. In addition, there is no single amount of humeral head translation beyond which instability or increased laxity can be diagnosed [36, 109, 110]. Further research is necessary before these types of instruments can be recommended for clinical practice. The use of ultrasound and stress radiographs have also been proposed as methods to measure joint translation; however, these methods are unreliable and, again, further testing is needed before they can be recommended for use in the clinical setting.
6.8 Laxity Testing
Patient guarding or apprehension is one of the main challenges associated with laxity testing of the shoulder in the office setting. With these maneuvers, it is required that the patient remains relaxed to allow the humeral head to translate appropriately during testing. While many of these tests can be performed in the sitting or supine position, several authors have noted that laxity testing with the patient in the supine position may produce the best results because the patient is generally more relaxed [111, 112]. Another challenge associated with laxity testing is the interpretation of the clinical findings. Although the end feel classification system derived by Cyriax and Cyriax [113] in 1947 has been used in the past (see Chap. 2), defining the quality of the end point in shoulder laxity testing is not practical since none of these qualities can be associated with any specific pathology, treatment or outcome. However, in the clinical setting, the reproduction of symptoms is often a strong indicator of the underlying diagnosis and may also direct the use of other provocative maneuvers.
6.8.1 Drawer Signs
Drawer signs can be used to assess anterior or posterior humeral head translation as long as the patient remains in a relaxed state throughout the maneuver. This is sometimes difficult in patients who present with overt instability where significant guarding and/or apprehension may be present. As mentioned above, it may be helpful to place the patient in the supine position to promote patient relaxation. When the patient is supine, it is also important to confirm that the humeral head is not supported by the examination table beneath as this will prevent posterior translation. On the other hand, posterior support of the scapula is advantageous since increased scapular rotation (internal or external rotation) may produce inaccurate results when attempting to manipulate the humeral head. Although the original developers of this maneuver recommended that the arm be placed between 80° and 120° of abduction, it is preferred to place the humerus in the approximate “loose pack” position [102, 114, 115] to (1) minimize the effects of proprioceptive muscle contraction (generated by increased capsular tension [16]), (2) to prevent scapular motion during testing, and (3) to more accurately assess true humeral head translation (Fig. 6.29). The glenohumeral resting position (or the “loose pack” position, discussed in Chap. 2) is debated; however, it is generally thought to occur between 55° and 70° of abduction within the scapular plane and with neutral rotation [116, 117].
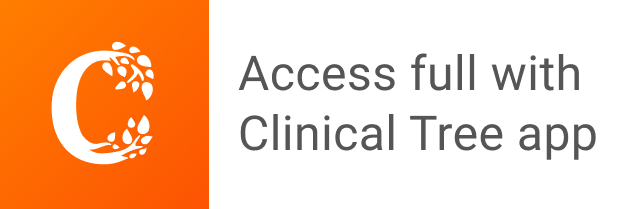