Exercise physiology is the identification and study of the physiologic mechanisms underlying physical activity and the body’s response and adaptation to exercise. Most training programs used by athletes, whether they are for strength or endurance, aim to change the underlying physiology for the benefit of sport competition or fitness. This chapter discusses the major concepts of exercise physiology to provide a background that sports medicine practitioners can draw upon when treating or training athletes.
Skeletal Muscle Physiology
The human body contains three types of muscle: smooth, cardiac, and skeletal. Training adaptations occur across all three types of muscle; however, this section will focus on skeletal muscle. Skeletal muscles support the body by working together to generate force across joints, leading to joint motion. Muscles also work to attenuate external forces in an effort to absorb shock and protect the joints.
Skeletal muscles are made up of different fiber types that have different properties such as rate of contraction, force generation, and resistance to fatigue ( Table 7-1 ). Skeletal muscle is made up of different fiber types. Fibers are currently classified by the myosin heavy chain isoform: one slow (type I) and three fast (IIA, IIB, and IIX). During the past several years, significant progress has been made in identifying signaling pathways that control expression of muscle fiber types. With the recently discovered microribonucleic acids encoded within myosin genes that regulate muscle gene expression and performance, a new individualized genetic differentiation may be possible.
I | IIa | IIx | IIb | |
---|---|---|---|---|
Contraction time | Slow | Medium fast | Fast | Very fast |
Size of motor neuron | Small | Medium | Large | Very large |
Resistance to fatigue | High | Fairly high | Intermediate | Low |
Activity | Aerobic | Long-term anaerobic | Short-term anaerobic | Short-term anaerobic |
Mitochondrial density | High | High | Medium | Low |
Muscle fibers are currently classified as type I, IIA, IIB, and IIX. In general, type I muscle fibers operate at a slower twitch rate and are responsible for longer term, endurance-type activities because of their ability to resist fatigue. Type IIA and IIB/X muscle fibers operate at a faster twitch rate and are responsible for high-force contractions that dominate during explosive, power maneuvers.
The true functional unit of the neuromuscular system is the motor unit. A motor unit is an alpha motoneuron (originating in the spinal cord) and all the muscle fibers that it innervates. Singular motoneurons innervate muscle fibers of same contractile type in an all-or-none fashion. Although a muscle will contain more than one or all types of motor units, the percentage of each fiber type within a muscle contributes to its function and fatigability. Motor units also can be classified as slow twitch or fast twitch, with the fast twitch units divided into fatigue resistant, fatigue intermediate, and fatigable. However, motor units can change in size in response to training and can also convert from one type to another. This plasticity of both contractile and metabolic properties allows the skeletal muscle system to adapt to different functional demands.
Structure of a Skeletal Muscle
Bundles of fascicles encased in connective tissue (epimysium) make up skeletal muscles. Each fascicle is surrounded by a layer of connective tissue (perimysium), which contains multiple muscle fibers. The muscle fiber, itself an individual skeletal muscle cell, is cylindrical in shape, multinucleated, and composed of bundles of myofibrils surrounded by an endomysium ( Fig. 7-1 ). These myofibrils contain thin and thick proteins that form repeating light and dark bands along the length of the myofibril, which give the muscle its striated appearance ( Fig. 7-2 ); these repeating sections are called sarcomeres and are arranged end to end within each myofibril.


Sarcomeres are the functional, contractile unit of skeletal muscle. Contractions are mediated through a dynamic interaction between actin and myosin, which are the contractile proteins. Spherical actin molecules in a helical arrangement form the thin protein structural lattice of the sarcomere. Each actin molecule contains a binding site protected by tropomyosin, a threadlike protein, and troponin, which acts as a stabilizing protein. Myosin molecules group together, each with a globular end, and form crossbridges, which are staggered toward opposite ends of the thick filament. These myosin molecules have a binding site with a high affinity for actin. This orientation of thick filaments at the center of the sarcomere provides the framework for sarcomere shortening and ultimately muscle contraction.
Physiology of Skeletal Muscle Contractions
Skeletal muscle contractions are a mechanical process driven by a neurochemical cascade. Several theories have been proposed about muscle contraction. The most widely accepted theory is the “sliding filament theory” proposed by Huxley and Hanson, in which the active shortening of the sarcomere and the muscle results from actin and myosin “sliding” past one another while retaining their original length. An action potential from the motoneuron is propagated and acetylcholine is released from the axon at the neuromuscular junction, increasing permeability to sodium and potassium ions and causing an end plate potential. This potential travels along the muscle cell membrane through the transverse tubule system throughout all the myofibrils. Calcium ions released from the sarcoplasmic reticulum quickly bind to troponin molecules on the thin contractile filament, resulting in the binding of tropomyosin to the exposed binding sites on actin, which allows the myosin crossbridges to bind with actin, pulling the actin filaments toward the middle of the sarcomere ( Fig. 7-3 ) and resulting in the filaments sliding past one another, causing muscle shortening and contraction. The collective shortening of myosin throughout the muscle is vital to force production and is termed the “power stroke.”

Calcium and adenosine triphosphate (ATP) are cofactors (nonprotein components of enzymes) required for the contraction of muscle cells. ATP supplies the energy, as previously described, but what does calcium do? Calcium is required by two proteins, troponin and tropomyosin, that regulate muscle contraction by blocking the binding of myosin to filamentous actin. The power stroke requires the hydrolysis of ATP, which breaks a high-energy phosphate bond to release energy, which leads to the release of the linkage between myosin and actin to prepare the myosin for another power stroke or for relaxation. If no new ATP is available, this linkage cannot separate, resulting in rigor mortis. Power stroke cycles continue until the concentration of calcium is no longer sufficient to cause the binding of actin and myosin.
Since the publication of the sliding filament theory by Huxley and Hanson in 1954, new mechanisms have been proposed for the mechanisms of muscle contractions. Several questions still remain, including enhancement of contractile force with stretch, depression of force with muscle contraction, and efficiency of force production during contraction. The winding filament model and the sticky spring model may both provide a means to assist in answering these questions by acknowledging the importance of the protein titin. Titin is activated by calcium influx and is wound upon the thin filaments by the crossbridges, which shorten but also rotate the thin filaments. Titin is also actively involved in force regulation of skeletal muscles, especially when muscles are stretched actively to long sarcomere lengths. These new models account for force enhancement during stretch and force depression during shortening, but additional study is needed to further validate these theories.
Force Production of Muscle
Muscle force can be increased or decreased by the recruitment of more or fewer motor units. For any motor task, many possible combinations of motor units could be recruited, and it has been proposed that a simple rule, the “size principle,” governs the selection of motor units recruited for different contractions. Motor units can be characterized by their different contractile, energetic, and fatigue properties, and it is important that the selection of motor units recruited for given movements allows units with the appropriate properties to be activated. The structural presence of titin also plays a role in the force generated by muscle.
Types of Muscle Contractions
Skeletal muscle contractions produce force and control joint movement and body control and are capable of different activities and demands through different types of muscle contraction. Isometric contractions occur when force is generated but no apparent change in total muscle length occurs. Concentric muscle contractions generate force that shortens the muscle. Eccentric muscle contractions occur when a force is generated as the muscle lengthens. Isotonic contractions produce muscle tension and joint movement against a constant load with a variable rate of movement. Isokinetic contractions have a constant rate of movement, which is maintained by varying the amount of muscle effort. Isokinetic efforts are rare in sport training but are utilized in the rehabilitation setting. In weight lifting or sport training, the two most common types of muscle contractions are the isometric, concentric activities where muscles are shortened in a voluntary fashion to lift a constant load (weight) and the eccentric contractions where the muscle lengthens against a constant load (negatives).
Although concentric muscle contractions are the contractions most people consider, eccentric contractions are probably more useful in the sports training and rehabilitation setting. During locomotion, eccentric contractions act to dissipate energy both as heat and as a shock absorber, reducing kinetic energy through a braking effect. The energy absorbed within the stretched muscle-tendon can then be stored as potential energy and returned, allowing the muscle-tendon complex to act as a spring. The muscle protein titin may be important in this springlike property of the muscle. Finally, the energy cost for eccentric contractions is unusually low, whereas the magnitude of forces produced is very high. Therefore as a response to eccentric loading, muscles gain strength, which makes eccentric loading well suited for both strength training and as a rehabilitation tool.
Energy Metabolism of Skeletal Muscle
Exercise results in an increased rate of ATP demand. To sustain muscle contraction, ATP needs to be regenerated at a rate complimentary to ATP demand. Three energy systems (phosphagen, glycolytic, and mitochondrial respiration) are available to synthesize ATP in muscle ( Fig. 7-4 ). The three systems differ in the substrates they use, the products they generate, their maximal rate of ATP regeneration, and their capacity for ATP regeneration.

Phosphagen Energy System
The phosphagen system is important for exercises that require short-term singular muscle contractions or a limited number of intense, near-maximal muscle contractions. Within the phosphagen system, the creatine kinase and adenylate kinase reactions both produce ATP, but the creatine kinase reaction has a much higher capacity for ATP regeneration given the large store of creatine phosphate (CrP) at rest. Another important feature is the adenylate kinase reaction, which produces adenosine monophosphate (AMP), which then activates two enzymes influential in glycolysis. AMP activates phosphorylase, which increases glycogenolysis and the rate of glucose-6-phosphate (G6P) production that becomes immediate fuel for glycolysis. AMP also activates phosphofructokinase, allowing an increase flux of G6P through glycolysis, which increases the rate of ATP regeneration.
It has been hypothesized that during the first 10 to 15 seconds of exercise, CrP is solely responsible for ATP regeneration. Near-complete recovery of CrP may take from 5 to 15 minutes, depending on the extent of CrP depletion, severity of metabolic acidosis, and characteristics of the muscle fibers utilized. Evidence is conflicting about the importance of oxygen during the resynthesis of CrP after high-intensity exercise. Some investigators suggest that CrP resynthesis is reliant on oxidative metabolism, whereas others found that after high-intensity exercise under ischemic conditions, glycolytic flux remained elevated for a short period. More recent work, however, supports the theory that glycolytic ATP production may contribute to CrP resynthesis during the initial fast phase of recovery after high-intensity exercise.
Glycolytic Energy System
When exercise continues for longer than a few seconds, the energy to regenerate ATP is derived primarily from blood glucose and muscle glycogen stores. This prolonged exercise increases the production of AMP. This production, coupled with the increases in intramuscular calcium and inorganic phosphate levels, cause increases in the phosphorylase reaction and the increased uptake of glucose by active muscle. The increased rate of G6P production from glycogenolysis and the increased glucose uptake provide a rapid source of fuel for several pathways that degrade G6P to pyruvate within the glycolytic pathway.
Traditionally it was thought that CrP was the sole fuel used at the initiation of glycolysis, with glycogenolysis occurring only once the CrP levels began to fall. However, research suggests that ATP resynthesis from glycolysis during 30 seconds of maximal exercise begins almost immediately with exercise. Maximal ATP regeneration capacity from glycolysis occurs when exercise at or above maximal oxygen uptake is performed for 2 to 3 minutes or as long as is sustainable by the athlete.
Lactate
During higher intensity exercise, an incomplete oxidation of glucose or glycogen occurs, with the excess pyruvate being converted to lactate via the lactate dehydrogenase reaction. For many years this leftover lactate was considered a waste product and a major cause of muscle fatigue or drop in performance. However, starting in the 1970s and continuing with the recent work of Brooks, this view has been challenged. It has now been shown that lactate is in fact beneficial during intense exercise. Production of lactate is essential in removing pyruvate to sustain glycolysis, and it is an oxidation-reduction process, reducing oxidized nicotinamide adenine dinucleotide (NAD + ) to reduced nicotinamide adenine dinucleotide phosphate (NADPH), which allows glycolysis to continue to produce ATP. Without lactate this process would slow and the amount of ATP, and thus the energy available for muscles, would drop. Most of the lactate formed during steady-state exercise is removed by oxidation, and only a small amount is converted to glucose. The lactate may also be “shuttled” from areas of high glycogenolysis to areas of high respiration via lactate shuttles. Although production of lactate initially was thought to occur only when anaerobic work was performed, it has been demonstrated that lactate is a valuable fuel during oxidative metabolism as well.
Mitochondrial Respiration (Oxidative System)
The connection between glycolysis and the mitochondria is complete when pyruvate and the electrons and protons from the reduction of NAD + to NADH are transferred into the mitochondria as substrates for mitochondrial respiration. The resynthesis of ATP involves the combustion of fuel in the presence of sufficient oxygen and occurs in the mitochondria. The fuel can be obtained from within the muscles (free fatty acids and glycogen), outside the muscle (fats), and in the form of blood glucose (ingestion of carbohydrates or release from the liver).
Carbohydrates in the form of glucose are the major fuel of the body during exercise. Humans gain most of their glucose from their diet. In the resting state, glucose is stored in the form of glycogen in both the liver and in skeletal muscle. The main fatty acid utilized at rest and during muscle contractions is palmitate (a 16-carbon fatty acid). Because the inner mitochondrial membrane is impermeable to fatty acids larger than 15 carbons, the fatty acids are transported via the carnitine shuttle into the mitochondria. Finally, muscle has an ample supply of amino acids (free amino acids) that can be utilized in a catabolic state when carbohydrate supply is too low. However, if carbohydrate supply is inadequate, protein catabolism and amino acid oxidation must occur. Intense exercise also increases this amino acid oxidation. Carbohydrate oxidation is the most efficient fuel in the production of ATP.
The energy systems respond differently to the diverse demands of sporting exercise. The nonmitochondrial (anaerobic) system is capable of responding immediately and is able to support high-power output via extreme muscle force. The phosphagen system is utilized for explosive, maximal efforts, which are very short in duration. The glycolytic pathway is used for activities that require sustained, intense efforts but are not maximal. Unfortunately, the anaerobic system is limited in its capacity, such that a reduction in power output will occur unless the demands can be supplemented by the aerobic system. The aerobic system is capable of responding quickly, yet it is incapable of meeting the initial high demands of intense exercise and is much better suited for longer efforts. The oxidative system is best for long-duration, low-intensity activities.
Muscular Response to Strength Training
Skeletal muscle exhibits superb plasticity in response to changes in functional demands. Chronic increases of skeletal muscle contractile activity, such as endurance exercise, lead to a variety of physiologic and biochemical adaptations in skeletal muscle, including mitochondrial biogenesis, angiogenesis, and fiber type transformation. These adaptive changes are the basis for the improvement of physical performance and other health benefits.
Muscles gain strength by getting larger (hypertrophy) and also by increasing skeletal muscle cell division (hyperplasia). The overload principle states that when a muscle is exposed to a stress or load that is greater than what it usually experiences, it will adapt so that it is able to handle the greater load. A metaanalysis of 17 studies concluded that mechanical overload resulted in increases in muscle mass, muscle fiber area (hypertrophy), and muscle fiber number (hyperplasia). Increases in fiber area were approximately twice as great as increases in fiber number.
As a skeletal muscle hypertrophies, contractile proteins are synthesized, and the muscle is therefore capable of producing more force. In response to heavy resistance training, type IIA fibers exhibit the greatest growth, whereas type IIB and type I exhibit the least amount of growth. Muscle hypertrophy is more common in fast-twitch than in slow-twitch muscles. Strength training leads to muscle hypertrophy, which increases muscle mass and typically occurs after weeks of such training. It appears that hyperplasia in skeletal muscle is greatest when certain types of mechanical overload, particularly stretch, are applied.
Muscle fibers within a motor unit have the same fiber type; however, skeletal muscles may contain several types of fibers based on demand of the muscle. This fiber type is genetically determined, but it may be altered with a training stimulus. Although the type of fiber cannot be changed, the amount of area taken up by a fiber type can be altered with training. Heavy resistance training causes increased type IIA and decreased type IIB fibers, whereas type I fiber composition in human skeletal muscle remains unchanged. In bench studies, structural and genetic characteristics of muscle fiber types have been modulated with fiber-specific stimulation ; it is unclear if this modulation will translate to human skeletal muscle.
Many of the initially observed strength gains of a weightlifting program are mostly due to neuromuscular adaptations. As exercise intensity increases and muscles begin to fatigue, the nervous system recruits larger motor units with higher frequencies of stimulation to provide the force necessary to overcome the imposed resistance. Early strength gains and increased muscle tension production from training result from a more efficient neural recruitment process.
Muscle Fatigue
Muscles that are used intensively show a progressive decline of performance that largely recovers after a period of rest; this reversible phenomenon is denoted as muscle fatigue. Many muscle properties change during fatigue, including the action potential, extracellular and intracellular ions, and many intracellular metabolites. Fatigue can be the result of peripheral causes, at the level of the skeletal muscle, or central causes.
Energy consumption of skeletal muscle increases dramatically with high-intensity exercise. The rate of energy need can exceed the aerobic capacity, forcing the muscles to rely on the anaerobic pathways for energy. Because high-intensity exercise leads to fatigue, it seems plausible that a relationship may exist between anaerobic exercise and fatigue. Anaerobic exercise leads to an accumulation of inorganic acids, mostly lactic acid, which dissociates into lactate and hydrogen ions. As previously discussed, lactate is a muscle fuel and therefore is probably not a factor in muscle fatigue, but the increase in hydrogen ions may be an important cause of muscle fatigue. Studies supporting this theory have shown a good temporal correlation between the decline of muscle pH and the reduction of force or power production. In addition, studies have demonstrated that acidification may reduce both the isometric force and the shortening velocity.
Peripheral fatigue can be a result of a reduced level of calcium during contractions, or it may be related to the contractile proteins themselves. During exercise, muscles depend on an ATP pump to return calcium to the sarcoplasmic reticulum; as exercise continues and the level of ATP drops, the ability to return calcium back to the sarcoplasmic reticulum drops and peripheral fatigue results. Another mechanism of peripheral fatigue occurs at the level of the crossbridges. It has also been demonstrated that the initial fall in muscle force is due to a reduction of the force generated by the individual crossbridges, whereas as fatigue progresses, a decline in the total number of crossbridges actually occurs. Finally, when muscles are fatigued fewer motor units are available, and thus reduced motor unit activation is a cause of fatigue.
Exercise performance is also dictated by the perception of effort of the brain and its inhibitory effects on central motor drive to the periphery. It is believed that these peripheral and central factors are closely linked, with the development of peripheral fatigue influencing the rate of development of “central” fatigue, operating through neural feedback pathways from working muscle to the motor control areas of the central nervous system.
Differentiation between central versus peripheral etiologies of muscle fatigue can be investigated via electrical stimulation. Muscles can be stimulated in a nonfatigued state and then again while fatigued, and if the characteristics of the contraction are different before and after fatigue, the likely cause is peripheral. If the contraction is reduced before fatigue, then the cause may be more central. Other etiologies of central fatigue are psychological and may be more difficult to determine. A major direction for future studies should be to identify the mechanisms that contribute to human fatigue in various activities and particularly during disease processes.
Neuromuscular Adaption to Exercise
Neuromuscular performance is determined not only by the quality and quantity of involved muscles but also by the ability of the nervous system to appropriately activate the muscles. These neural adaptive changes in response to training are referred to as neural adaption. These neural factors are important for increases in strength early in resistance training, whereas muscular hypertrophy of trained muscles develops after prolonged resistance training.
Resistance training–induced adaptations are supraspinal in nature and include increased excitation and changes in the organization of the motor cortex. Such adaptations can influence the manner in which muscles are recruited for functional tasks. Changes at the neuromuscular junction, which are associated with functional alterations in transmission, may lead to enhanced neuromuscular transmission. These adaptations can improve the activation of muscles and are likely the result of muscle training. Increased levels of muscle activation and subsequent increase in force achieved by increases in individual firing at the motor unit leads to the recruitment of more motor units.
In addition to these neural factors, the amount of force that a muscle can exert is influenced by the number and size of fibers and the fiber type. Heavy resistance exercise results in increases in strength and changes in both neuromuscular function and muscle morphology. Finally, neural adaptations are found in the level of coactivation of antagonist muscles and changes in the synergistic patterns of muscle activations, which could also contribute to maximization of force generation.
In contrast to resistance training, the neural response to endurance training involves strategies to improve efficiency, such as reducing the number of motor units needed to maintain force and increasing the activation of synergistic muscles. Endurance training results in increased capillary density and decreased muscle cross-sectional area to facilitate oxygen delivery to tissues. In addition, endurance training leads to an increase in cellular mitochondrial content and oxidative enzyme activity, also increasing aerobic capacity. These changes require 8 to 12 weeks of training to realize. The net results of long-term endurance training are increased metabolic capacity of muscle, increases in muscular force output, and muscle hypertrophy.
Delayed-Onset Muscle Soreness
The demands of the training stimulus causes microdamage to muscles, and their ability to recover and regenerate is part of the training process. Muscle soreness may occur during this period, which peaks 24 to 48 hours after a strenuous bout of training and typically resolves by 96 hours. This phenomenon is known as delayed-onset muscle soreness (DOMS). DOMS is typically experienced by all persons regardless of fitness level and is a normal physiologic response to increased exertion and the introduction of unfamiliar physical activities. The resulting sensation of pain and discomfort can impair physical training and performance, and thus the etiology and prevention of DOMS is of interest to athletes and trainers.
More muscle damage may occur with higher intensity or unfamiliar exercises, predominantly eccentric loading exercises. Other factors that may be involved include muscle stiffness, contraction velocity, fatigue, and angle of contraction. Some evidence indicates that fast-twitch muscle fibers are more susceptible to eccentric induced injury. The initial injury to the muscles is a mechanical disruption of the sarcomeres that proliferates a secondary inflammatory response, releasing prostaglandin E2 and leukotrienes, which gives the sensation of pain and contributes to swelling as a result of increased vascular permeability. The initial injury pattern is sporadic throughout the muscle, and the sarcomere damage does not extend the length of the myofibril, nor does it extend across a whole muscle fiber. Thus the muscle injury pattern of DOMS differs from that of an acute muscle strain, which occurs as an isolated disruption of the muscle-tendon junction extending across the fibers.
Can the symptoms and functional consequences of DOMS be mitigated or prevented? It would seem reasonable that if DOMS is thought to be an inflammatory problem and is mediated by prostaglandins, then the use of nonsteroidal antiinflammatory drugs (NSAIDs) or the application of ice would help reduce or prevent DOMS. However, studies show mixed results with NSAIDs, and because NSAIDs are not without risk, further data are needed to recommend the use of NSAIDs to treat DOMS. Ice offers an analgesic effect but little sustained benefit. Muscle stretching does not appear to produce clinically important reductions in DOMS in healthy adults. Antioxidants are not effective and may actually delay muscle recovery. However, studies do show that massage, as well as massage plus the use of compressive clothing, is of benefit in reducing symptoms of DOMS. Finally, whole-body vibration prior to exercise may be effective for reducing DOMS.
Strength Training in Youth Athletes
Strength training in young athletes has been controversial. Some of the controversy originated from misconceptions about benefits of training and the risk to the skeletally immature. Previously, it was thought that prepubertal athletes could not benefit from weight training because of low levels of gonadal hormones and that youth may lose flexibility and range of motion with strength training. Finally, it was thought that strength training exposes the growth plates of young athletes to excessive risk of injury. Recently, however, research has demonstrated that well-designed resistance training programs can enhance the strength of children and adolescents beyond what is expected with normal maturation. Many leading sports organizations now recognize the importance of muscular strength during childhood and adolescence.
Strength gains of up to 75% can be observed in young athletes after an 8- to 12-week training program. Lighter loads and a greater number of repetitions (10 to 15) are recommended to increase strength. These training-induced changes in youth are predominately due to neural adaptations as opposed to the muscle hypertrophy seen in adults. Strength training in youth also has the potential to offer other many health benefits for children. Improved bone health, body composition, reduction of cardiovascular risk factors, and decreased risk of sports injury results from strength training. Therefore it would appear that the benefits outweigh the risks and that properly designed and supervised programs are safe and effective for young athletes.
Effects of Aging on Skeletal Muscle
Aging has been associated with loss of muscle mass, which is referred to as sarcopenia. This decrease begins around age 50 years but becomes more dramatic after 60 years. Aging negatively affects power, strength, and endurance in skeletal muscles. Recently, muscle power has been found to be a better predictor of functional performance than muscle strength. Loss of power contributes to a decrease in short-term anaerobic performance and is directly related to the increase in falls among older people and impairment in the performance of daily activities. The etiology of this loss of power is from both a loss of muscle mass and a slowing in the firing of motor neurons and maximal shortening velocity. The loss of strength is directly related to the loss of muscle mass. Sarcopenia accounts for more than 90% of age-related strength decrease. Other factors involved in strength reduction are decreases in the tension of muscle tissue due to aged myofibrils or a decrease in the number of functioning crossbridges. Despite the negative effects of aging, strength training can improve power and strength, reversing some of the decrement and leading to improved functionality in older adults. High-velocity resistance training and increasing lower extremity strength has been shown to be the best way to achieve this goal.
Core Strength and Neuromuscular Training
Core stability is an important component in maximizing efficient athletic function, as well as in preventing injury. Core stability can be defined as the “ability to control the position and motion of the trunk over the pelvis to allow optimum production, transfer and control of muscle activity.” The core consists of the muscles of the trunk and pelvis but also the neural, osseus, and ligamentous elements. The stability is dependent not only on muscular strength but also on proper sensory input that alerts the nervous system about interaction between the body and the environment, providing constant feedback and allowing for refinement in movement. These proprioceptive abilities have a positive impact on reducing injuries, and exercise programs targeting the enhancement of these abilities may prevent injuries. Some evidence indicates that balance training or multifaceted training programs are effective in preventing lower limb injuries, such as ankle sprains and anterior cruciate ligament tears among young adults when they participate in ball sports. Finally, after injuries have occurred, rehabilitation of deficits may prevent future injuries. To date, however, the most effective training program and the optimal frequency and duration of these sessions have yet to be determined, and more research is needed.
Hormonal Adaption to Exercise
Many changes that occur in response to exercise are mediated by hormones. Growth hormone, endorphins, and antidiuretic hormone from the pituitary assist in the performance and recovery of muscles. Adrenal hormones such as catecholamines, glucocorticoids, and mineralocorticoids are responsible for most of the key changes needed in exercise, although many other hormones are also involved. Pancreatic and gonadal hormones also play a key role ( Table 7-2 ).
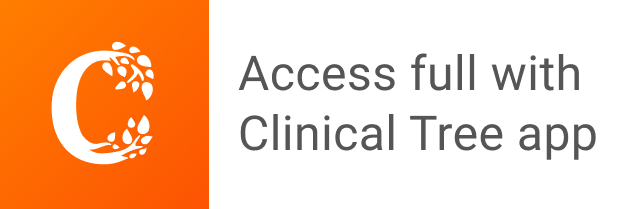