Embryology and Development of the Neuromuscular Apparatus
José A. Morcuende
James O. Sanders
INTRODUCTION
The development of an adult organism from a single cell is an unparalleled example of integrated cell behavior. The single cell divides many times to produce the trillions of cells of the human organism, which form structures as complex and varied as the eyes, limbs, heart, or the brain. This amazing achievement raises multitude of questions. How the body’s tissues and organs are formed? How do the different patterns form in the embryo that tells different parts what to become? How individual cells become committed to particular development fates? Increased knowledge in developmental biology comes from the understanding of how genes direct those developmental processes. In fact, developmental biology is one of the most exciting and fast-growing fields of biology and has become essential for understanding many other areas of biology and medicine.
Embryology at the level of gross anatomy and microscopic anatomy is fairly well described. Manipulation of experimental animals, mainly the chick and mouse, has provided insights into the relationship of tissues involved in normal growth and differentiation. Molecular mechanisms underlying developmental events are being discovered. An integration of the approaches of genetics, molecular biology, developmental biology, and evolutionary biology is taking place, resulting in an explosion in our understanding of the importance of individual genes and interactions of cells and tissues in specifying development of complex organisms from single cells. One of the major reasons for the synthesis and complementariness of these varying disciplines is the existence of homology, both within organisms and between species. It turns out that genes and their gene products are often very similar in structure and function in fruit flies, chickens, mice, and men. In complex organisms, the same gene is often used at different times in development and in different areas of the body to perform similar functions.
This chapter describes the early stages of embryonic development, followed by the descriptive anatomy of limb development and the formation of the vertebral column. It also examines bone formation and growth and emphasizes the progress in the understanding of the cellular and molecular mechanisms involved in these aspects of development. Concluding each section are observations that relate developmental anatomy to the clinical problems faced by orthopaedic surgeons. Finally, a section on growth in pediatric orthopaedics will provide a framework of understanding growth and development’s effects on the musculoskeletal system.
DEVELOPMENTAL ANATOMY OF EARLY EMBRYOGENESIS AND ORGANOGENESIS
Embryogenesis has been traditionally divided into the embryonic period and the fetal period. The embryonic period is considered from fertilization to the end of the first trimester. During this period, the body plan is completed and all major organs are established. The stages of the embryonic period include fecundation, cleavage, gastrulation, neurulation, and organogenesis. By the 12th week of gestation, the organism shape is fully formed and the remaining of the gestation will involve the growth and the maturation of the organ functions.
Creating Multicellularity.
The first stage of development after fertilization is a series of cleavage divisions in which the zygote divides in an ordered pattern to produce a ball of much smaller cells, called blastula, and this starts the production of a multicellular organism. Cleavage is a very well-coordinated process under genetic regulation. The specific type of cleavage depends upon the evolutionary history of the species and on the mechanism used to support the nutritional requirements to the embryo. The pattern and symmetry of cell cleavage particular to a species is determined by the amount and distribution of the cytoplasm (yolk), and by those factors in the cytoplasm influencing the angle of the mitotic spindle and the timing of its formation. In most species (mammals being the exception), the rate of cell division and the placement of the blastomeres with respect to one another is completely under
the control of proteins and mRNA stored in the mother oocyte. The zygote DNA is not used in early cleavage embryos. In addition, the differential cellular cleavage provides the embryo with axis information, dividing the cell in an animal pole (where the nucleus is frequently found) and a vegetal pole.
the control of proteins and mRNA stored in the mother oocyte. The zygote DNA is not used in early cleavage embryos. In addition, the differential cellular cleavage provides the embryo with axis information, dividing the cell in an animal pole (where the nucleus is frequently found) and a vegetal pole.
In mammals, the protected uterine environment permits an unusual process of early development. It does not have the same need as the embryos of most other species to complete the early stages rapidly. Moreover, the development of the placenta provides for nutrition from the mother, so that the zygote does not have to contain large stores of material such as yolk. Thus, cleavage has several specific characteristics. First, it is a relatively slow process. Each division is about 12 to 24 hours apart. A frog egg, for example, can divide into 37,000 cells in just 43 hours. Second, there is a unique orientation of the cells with relation to one another. The first cleavage is a meridional division, but in the second division, one pair of cells divides meridionally and the other equatorially (Fig. 1-1). This type of cleavage is called rotational cleavage (1). Third, there is an asynchrony in the early divisions. Cells do not divide at the same time. Therefore, embryos do not increase evenly from 2- to 4- to 8-cell stages but frequently contain odd number of cells. Fourth, the zygotic genome is activated early during cleavage divisions to produce the proteins needed for the process to occur (2). Finally, the most crucial difference with other species is the phenomenon of compaction. At 8-cell stage, blastomeres form a loose arrangement of cells, but after the third division, the cells cluster together and form a compact ball with the outside cells stabilized by tight junctions and the inner cells developing gap junctions which enables the passing of small molecules and ions between cells (Fig. 1-2).
Up to the 8-cell stage, the embryo is remarkably adaptable, and each of its cells can form any part of the later embryo or adult. One example is seen in the development of a pair of identical twins from a single fertilized egg. Similarly, this embryonic cell potential can be demonstrated experimentally by using chimeras. These are animals made by combining individual cells from early embryos of genetically different strains of animals and then the reaggregated cells implanted in foster mothers. Analysis of the genetic composition of the tissues of the developed animal shows that the single cells from the four-cell stage can participate in forming many different parts of the animal; they are said to be totipotent (3, 4).
In mammals, the next stage in development is the generation of the cells that will form the placenta and the membranes that surround the developing embryo. The cells of the compacted embryo divide to produce a 16-cell morula. This morula consists of a small group (one or two) of internal cells surrounded by a larger group of external cells (Fig. 1-2D) (5). The
position of a cell at this stage determines whether it will form extraembryonic structures or contribute to the embryo proper. Inner cells will form the embryo and most of the external cells the trophoblast. This structure will enable the embryo to get oxygen and nourishment from the mother and will secrete hormones and regulators of the immune response so that the mother will not reject the embryo. Experimentally, this separation of cell activities has been shown also with chimeras. Cells from different strains of mouse can be arranged so that the cells of one strain surround the cells of the other strain. The development of such cell aggregates shows that only the cells on the inside contribute to the mouse development (6). By the 64-cell stage, the inner cell mass and the trophoblast have become separate cell layers, neither of which contributes cells to the other group. Thus, the distinction between these two cell types represents the first differentiation event in mammalian development.
position of a cell at this stage determines whether it will form extraembryonic structures or contribute to the embryo proper. Inner cells will form the embryo and most of the external cells the trophoblast. This structure will enable the embryo to get oxygen and nourishment from the mother and will secrete hormones and regulators of the immune response so that the mother will not reject the embryo. Experimentally, this separation of cell activities has been shown also with chimeras. Cells from different strains of mouse can be arranged so that the cells of one strain surround the cells of the other strain. The development of such cell aggregates shows that only the cells on the inside contribute to the mouse development (6). By the 64-cell stage, the inner cell mass and the trophoblast have become separate cell layers, neither of which contributes cells to the other group. Thus, the distinction between these two cell types represents the first differentiation event in mammalian development.
Organizing the Embryonic Cells to Form Tissues and Organs.
In human beings, implantation begins 1 or 2 days after the blastocyst enters the uterus, approximately on day 9 (Fig. 1-3A). At the time of implantation, the exposed surface of the uterine lining, the endometrium, is a single-layered epithelial sheet, which forms numerous tubular glands. Having adhered to the epithelium, the trophoblastic cells penetrate it and erode it (Fig. 1-3B). The endometrium responds by a dramatic increase in vascularity and capillary permeability, the so-called decidual reaction (Fig. 1-4). These processes are apparently mediated by estrogens produced by the blastocyst and estrogen receptors in the wall of the uterus. In addition, the trophoblast initiates the secretion of chorionic gonadotropin, which will maintain the production of progesterone by the ovaries, which is essential for the maintenance of the pregnancy. HCG is detectable in the blood and urine and serves as the basis of pregnancy tests.
The next phase of development—the gastrulation— involves a remarkable process in which the ball of cells of the blastula turns itself into a multilayered structure and rearranges to form the three embryonic tissue layers known as endoderm, ectoderm, and mesoderm. In addition, during gastrulation, the body plan of the organism is also established. Gastrulation thus involves dramatic changes in the overall structure of the embryo, converting it into a complex three-dimensional structure.
The mechanics of gastrulation in mammals are not well understood. In sea urchins and insects, the phenomenon of gastrulation is like what happens if a ball is punctured and then kicked: the ball collapses and the inner surface on one
side makes contact with the other side, making a large dimple. In the embryo, by a complicated invagination, a large area of cells on the outside of the embryo is brought to lie inside it. Subsequent development depends on the interactions of the outer ectoderm, middle mesoderm, and inner endoderm layers of cells. The ectoderm will give rise to the epidermis of the skin and the nervous system; the mesoderm will give rise to connective tissues including the bones, muscle, and blood; and the endoderm will give rise to the lung and the lining of the gut and associated organs.
side makes contact with the other side, making a large dimple. In the embryo, by a complicated invagination, a large area of cells on the outside of the embryo is brought to lie inside it. Subsequent development depends on the interactions of the outer ectoderm, middle mesoderm, and inner endoderm layers of cells. The ectoderm will give rise to the epidermis of the skin and the nervous system; the mesoderm will give rise to connective tissues including the bones, muscle, and blood; and the endoderm will give rise to the lung and the lining of the gut and associated organs.
In addition, during gastrulation, the cells are positioned according to the body plan appropriate to the species, and there is a process of differentiation of the functional characteristics required of each part of the body plan. Specification of the axes in mammals does not involve any maternal component. The dorsoventral (DV) axis is established by the interaction between the inner cell mass and the trophectoderm, while the anterior-posterior (AP) axis may be set only at implantation. The generation of the left-right asymmetry is under genetic control. This vertebrate body plan will be maintained thereafter as the embryo grows.
The movements of gastrulation involve the entire embryo. Cell migration in one part of the embryo must be intimately coordinated with other cell movements occurring simultaneously elsewhere. However, gastrulation depends on a relatively simple repetition of basic cell activities. Cells can change their shape by extending or contracting. They can group or separate by forming or breaking their adhesions to neighboring cells or to the extracellular matrix. They can secrete extracellular matrix that constrains or guides their location or movement. These activities, together to cell proliferation, underlie almost all-morphogenetic activities during gastrulation. The special problem posed in early embryonic development is to understand how these and other elementary cell activities are coordinated in space and time.
Interestingly, recent experiments have suggested that the maternal and paternal genomes (imprinting) have different roles during mammalian gastrulation. Mouse zygotes can be created that have only sperm-derived or oocyte-derived chromosomes. The male-derived embryos die without embryo proper structures but with well-formed chorionic structures. Conversely, the female-derived embryos develop normally but without chorionic structures (7, 8 and 9). Therefore, the maternal and paternal genomic information may serve distinct functions during early development.
Early Organogenesis in Vertebrate Development: Neurulation and Mesoderm Segmentation.
During gastrulation, the germ layers—ectoderm, mesoderm, and endoderm—move to the positions in which they develop into the structures of the adult organism. The AP body axis of the vertebrate embryo emerges, with the head at one end and the future tail at the other. During the next stage of development, the main organs of the body begin to emerge gradually (10). A major set of interactions takes place between the mesodermal cells and the ectoderm in the dorsal midline (Hensen’s node) so that the ectoderm cells layer will form the nervous system (Fig. 1-5). At the same time, the mesoderm on either side of the middle breaks up into blocks of cell to form the somites, a series of repeated segments along the axis of the embryo (Fig. 1-6) (11). The interactions between the dorsal
mesoderm and its overlying ectoderm are one of the most important interactions of all development. The action by which the flat layer of ectodermal cells is transformed into a hollow tube is called neurulation (Fig. 1-7). The first indication of neurulation is a change in cell shape in the ectoderm. Midline ectodermal cells become elongated, whereas cells destined to form the epidermis become flattened. The elongation of the cells causes this region to rise above the surrounding ectoderm, thus creating the neural plate. Shortly thereafter, the edges of the neural plate thicken and move upward to form the neural folds that subsequently will fuse to form the neural tube beneath the overlying ectoderm. The formation of the neural tube does not occur simultaneously. It starts near the anterior end of the embryo and proceeds in both directions—anteriorly and posteriorly. The two open ends are called anterior and posterior neuropores (Fig. 1-8). In mammals, failure to close the anterior neuropore results in anencephalia, and the posterior neuropore in spina bifida. Neural tube defects (NTDs) can now be detected during pregnancy by ultrasonography and chemical analysis of the amniotic fluid.
mesoderm and its overlying ectoderm are one of the most important interactions of all development. The action by which the flat layer of ectodermal cells is transformed into a hollow tube is called neurulation (Fig. 1-7). The first indication of neurulation is a change in cell shape in the ectoderm. Midline ectodermal cells become elongated, whereas cells destined to form the epidermis become flattened. The elongation of the cells causes this region to rise above the surrounding ectoderm, thus creating the neural plate. Shortly thereafter, the edges of the neural plate thicken and move upward to form the neural folds that subsequently will fuse to form the neural tube beneath the overlying ectoderm. The formation of the neural tube does not occur simultaneously. It starts near the anterior end of the embryo and proceeds in both directions—anteriorly and posteriorly. The two open ends are called anterior and posterior neuropores (Fig. 1-8). In mammals, failure to close the anterior neuropore results in anencephalia, and the posterior neuropore in spina bifida. Neural tube defects (NTDs) can now be detected during pregnancy by ultrasonography and chemical analysis of the amniotic fluid.
![]() FIGURE 1-6. Scanning electron microscopy showing the neural tube and the well-formed somites with paraxial mesoderm that has not yet separated into distinct somites. (Courtesy of KW Tosney.) |
The process of neurulation is intimately linked to changes in cell shape generated by the cytoskeleton (microtubules and microfilaments). Differential cell division seen in different regions of the neural plate would also contribute to the size and shape of this region. In addition, those cells directly adjacent to the notochord and those cells at the hinges of the neural groove will also help to mold the neural tube. Separation of the neural tube from the ectoderm that will form the skin requires changes in cell adhesiveness. While molecules that can induce neural tissue, such as noggin protein, have been identified, induction of neurulation is due to inhibition of bone morphogenetic protein (BMP) activity. Positional identity of cells along the AP axis is encoded by the combinatorial expression of genes of the four Hox complexes.
The cells at the dorsal-most portion of the neural tube become the neural crest. These cells will migrate throughout the embryo and will give rise to several cell populations.
Although derived from the ectoderm, the neural crest has sometimes been called the fourth germ layer because of its importance. It gives rise to the neurons and supporting glial cells of the sensory, sympathetic, and parasympathetic nervous systems; the melanocytes of the epidermis; and the cartilage and connective tissue components of the head. Although not well understood, the mechanisms of neural crest migration are not random but rather follow precise pathways specified by the extracellular matrix. Differences in adhesiveness between the anterior and posterior halves of the somites result in neural crest being prevented from migrating over the posterior halves. Thus, presumptive dorsal ganglia cells collect adjacent to anterior halves, giving them a segmental arrangement.
Although derived from the ectoderm, the neural crest has sometimes been called the fourth germ layer because of its importance. It gives rise to the neurons and supporting glial cells of the sensory, sympathetic, and parasympathetic nervous systems; the melanocytes of the epidermis; and the cartilage and connective tissue components of the head. Although not well understood, the mechanisms of neural crest migration are not random but rather follow precise pathways specified by the extracellular matrix. Differences in adhesiveness between the anterior and posterior halves of the somites result in neural crest being prevented from migrating over the posterior halves. Thus, presumptive dorsal ganglia cells collect adjacent to anterior halves, giving them a segmental arrangement.
The formation of mesodermal structures is not subsequent to the neural tube but occurs simultaneously. The brain and spinal cord must develop in the correct relationship with other body structures, particularly the mesoderm. Five regions of mesoderm can be identified at the neurula-stage embryo (Fig. 1-9). The chordamesoderm will generate the notochord, a transient organ whose functions include inducing neural tube formation and establishing the body axis. The dorsal (somitic) mesoderm will produce many of the connective tissues of the body. The intermediate mesoderm will form the urinary system and genital ducts. The lateral plate mesoderm will give rise to the heart, blood vessels, and blood cells, and the body lining cavities. Lastly, the head mesoderm will contribute to the connective tissues and muscles of the face.
At the neural stage, the body plan has been established and the regions of the embryo that will form limbs, eyes, heart, and the other organs have been determined. But although the positions of various organs are fixed, there is no overt sign yet of differentiation. The potential to form a given organ is now confined to specific regions. Each region has, however, considerable capacity for regulation, so that if a part of the region is removed a normal structure can still form. In later sections, limb and axial skeleton formation will be discussed in more detail.
Conceptual Insights of Embryogenesis and Early Organogenesis.
Development is essentially the emergence of organized and specialized structures from an initially very simple group of cells. Thus, the cells of the body, as a rule, are genetically alike (they all have the same DNA content) but phenotypically different—some are specialized as muscle, others as neurons, and so on. During development, differences are generated between cells in the embryo that lead to spatial organization, changes in form, and the generation of different cell types. All these features are ultimately determined by the DNA sequence of the genome. Each cell must act according to the same genetic instructions, but it must interpret them with regard to time and space.
Multicellular organisms are very complex, but they are generated by a limited repertoire of cell activities. As an artist moves from one part of a sculpture to another to achieve first the overall’s figure shape, and then the specific anatomic features using a selected number of instruments over and over again, nature also displays a comparable economy in choosing the processes and molecular tools. The key to understanding development lies in cell biology, in the processes of signal transduction, and in the control of gene expression that result in changes of cell state, movement, and growth. The single most important fact in development is based on the surprising finding that the developmental control genes are maintained through evolution. Thus, for many genes discovered in the invertebrate systems, homologue genes have been identified in vertebrates and they have similar developmental roles in species ranging from the fruit fly to fish to mouse to human.
It is convenient to distinguish three main developmental processes, even though they overlap with, and influence, one another considerably. These are the emergence of pattern, cell differentiation, and change in form or morphogenesis.
Pattern Formation.
Pattern formation is the process by which spatial and temporal arrangements of cell activities are organized within the embryo so that a well-defined structure develops. Pattern formation is critical for the proper development of every part of the organism. In the developing limb, for example, pattern formation enables the cells to know whether to make the upper arm or the fingers, and where the muscles should form.
Pattern formation in many animals is based on a mechanism where the cells first acquire a positional identity, which determines their future behavior. The ability of cells to sense their relative positions within a limited population of cells and to differentiate according to this position has been the subject of intense research. Interestingly, pattern formation in many systems has similar principles, and more striking similar genes. Many of the so-called homeotic genes that determine segment identity in Drosophila have turned up in vertebrates and appear to play similar roles in segmentation of structures such as the brain or the vertebral column.
Homeotic genes are like embryonic switches, analogous to switches of railroad yards that directed trains into one path rather than another. Homeotic genes are involved in specifying regional identity along the AP axis. The name comes from the fact that mutations in some of these genes result in what is called a homeotic transformation, in which one body structure replaces another. For example, in mice in whom Hoxd11 is mutated, anterior sacral vertebrae are transformed into lumbar vertebrae. Homeotic genes in all systems work similarly: they code for proteins called transcription factors that control gene expression. In vertebrates and Drosophila, the order of homeotic genes on the chromosome corresponds to their temporal and spatial expression on the AP axis of the embryo.
Cell Differentiation.
Cell differentiation is the process in which cells become structurally and functionally different from each other, ending up as distinct types as muscle, bone, or cartilage. Since each cell of the organism has the same genetic material, the achievement and persistence of the differentiation state depends on a series of signals that ultimately control the transcription of specific genes. In humans, the zygote gives rise to about 250 clearly distinguishable types of cells. One of the major goals of developmental biology is to discover how these differences emerge from the fertilized oocyte.
In any organism, differentiation leads to the production of a finite number of discrete kinds of cells, each with its peculiar repertory of biochemical activities and possible morphological configurations. When cells achieve a distinctive state of differentiation, they do not transform into cells of another type. Differentiation leads to a stable, irreversible set of cellular activities. At the organ level, once an embryonic part is capable of realizing its prospective fate in the absence of the conditions that established that capability, it is said to be determined. Determination is thus a step that limits the subsequent development of the part to a specific tissue and cellular differentiation.
Pattern formation and cell differentiation are very closely interrelated as we can see by considering the difference between the upper and lower extremities. Both contain the same tissues—muscle, cartilage, bone, and so on—yet the pattern in which they are arranged are different. It is essentially pattern formation that makes human beings different from rabbits or chimpanzees.
Morphogenesis.
Although vertebrate morphogenesis— change in form—is far from completely understood, developmental biology findings support that the same family of molecules and pathways that guide the earliest stages of embryogenesis—setting up such basic elements of body pattern as the head-to-tail and DV axis—also help out in morphogenesis. What is more, these molecules and pathways have been conserved over the course of evolution. Morphogenesis relies on a rather restricted number of cellular activities and encompasses the formation of all tissues and organs from the first embryonic tissue layers to the finished limb, spine, or brain. However, before any tissue or organ can form, earlier steps must occur, steps that tell cells who they are and what tissues they should form. Those early steps take place in the “control room” for development, and morphogenesis is then what happens on the “factory floor”—the actual assembly of the tissues and organs that make up the organism. In addition, spatial patterns of cell proliferation, folding of cell groups, rearrangement of cells, and cell migration make important contributions to morphogenesis, the process that shapes the embryo. Finally, as the embryo develops, cells become different, and this process culminates in the specialization of cells for particular functions. Therefore, during development, morphogenesis give rise to structures appropriate to their position within the embryo and, within these structures, the differentiation of individual cells and their interactions are spatially ordered.
Clinical Significance.
Broadly defined, birth defects or congenital abnormalities occur in 6% of all live births. Twenty percent of infant deaths are due to congenital anomalies. About 3% of newborns have significant structural abnormalities. At present, the cause of approximately 50% to 60% of birth defects is unknown. Six to seven percent are due to chromosomal abnormalities. Specific gene mutations cause 7% to 8%. Environmental teratogens are responsible for 7% to 10% of defects. Combined genetic predisposition with environmental factors causes the remaining 20% to 25% of congenital abnormalities.
Starting from a single cell, the embryo can spawn all the new cells and tissues needed to provide an organism with its correct complement of organs. Many of the molecules and pathways known to control cell differentiation and growth during organ formation in the embryo do not become obsolete in the adult. They do help maintain and repair tissues and regulate their response to external environment signals. Some of these proteins are or will soon be in clinical use such as erythropoietin, which trigger red blood cell production, platelet-derived growth factor (PDGF) for diabetic skin ulcers, or BMPs for bone and cartilage regeneration. Finally, in malignant disease, the control of cell activities such as proliferation, differentiation, and migration appears to break down. An understanding of the way in which cell behavior is coordinated in embryos could therefore give insights into bone and cartilage regeneration and cancer biology.
DEVELOPMENTAL ANATOMY OF THE LIMB
At 26 days after fertilization, the upper limb is evident as a slight elevation on the ventrolateral body wall at the level of the pericardial swelling. The lower limb elevation appears 2 days later just caudal to the level of the umbilical cord and develops similarly, but slightly later than the upper limb (Fig. 1-10). At this time the neural tube is closed, all somites are present, and the anlage of the vertebrae and intervertebral discs are present. The limb bud initially consists of loose mesenchymal tissue enclosed in an epithelial ectodermal sheath. The limb bud is formed from mesenchymal cells of the lateral plate and then augmented by cells from the adjacent somites. The skeletal elements and tendons develop from the lateral plate mesenchyme, while limb muscle arises from somitic mesenchymal cells that migrate into the limb bud.
This mesenchymal swelling is covered by ectoderm, the tip of which thickens and becomes the apical ectodermal ridge (AER) (Fig. 1-11). Underlying the AER are rapidly proliferating, undifferentiated mesenchymal cells which are called the progress zone (PZ). Proliferation of these cells causes limb outgrowth. Cells begin to differentiate only after leaving the PZ. The interaction between the AER and the undifferentiated mesenchymal cells underlying it is crucial for limb development. Experimental procedures on chick embryos reveal the following about the limb bud mesenchyme: (a) if removed, no limb develops; (b) when grafted under the ectoderm at a location other than the normal limb area, an AER is induced and a limb will develop; and (c) lower limb mesoderm will induce leg formation, when placed under an upper limb AER. Grafting experiments with the AER reveal that: (a) AER removal aborts further limb development. The later in limb development the AER is removed the less severe is the resulting limb truncation (limb elements develop from proximal to distal); (b) An extra AER will induce a limb bud to form supernumerary limb structures; (c) Nonlimb mesenchymal cells placed beneath the AER will not result in limb development and the AER withers (12).
The implications of these experiments are that the AER is necessary for the growth and development of the limb, while the limb bud mesenchyme induces, sustains, and instructs the AER. In addition to biochemical influence on the PZ, the tightly packed columnar cells of the AER perform a mechanical function directing limb shape by containing these undifferentiated cells in a dorsoventrally flattened shape. The length of the AER controls the width of the limb as well. When all limb elements are differentiated, the AER disappears.
![]() FIGURE 1-10. Timing of the appearance of limb features. (Reproduced from Thorogood P. Embryos, genes and birth defects. West Sussex, UK: John Wiley & Sons, Ltd, 1997:350, with permission.) |
![]() FIGURE 1-11. Scanning electron photomicrograph of an early chick forelimb bud with the AER at the tip of the limb bud. (Courtesy of KW Tosney.) |
The three axes of limbs—AP (thumb to small finger), DV (back of hand to palm), and proximal-distal—are specified very early in limb development. The AP and DV axes are fixed before morphological differentiation of limb components occurs. The PD axis is determined as the limb grows out. The AP axis is set first, followed by the DV axis and then the PD axis. This has been shown by rotation of transplanted limb buds’ from their normal position and finding, at different stages, that the limb bud retained the axis orientation of its original limb position or developed the orientation of the host bud (9, 13, 14).
AP axis determination is under the control of an area of tissue in the posterior aspect of the limb bud called the zone of polarizing activity (ZPA) or polarizing region. If this tissue is grafted onto the anterior aspect of a limb bud, a duplication of digits in a mirror image to the normally present digits occurs (15). Cells for the new digits are recruited from the underlying mesoderm and the distal part of the limb widens, as does the AER. If less tissue from the polarizing region is grafted, fewer new digits develop (16). This and other experiments suggest a morphogenic gradient of a diffusible signal originating from the ZPA determines AP axis. This will be discussed in the section on the molecular biology of limb development.
The DV axis is under the control of both the mesoderm and ectoderm of the limb bud at different stages of development. The mesoderm specifies the axis initially, but very early after limb bud formation the ectodermal orientation becomes preeminent. If the ectoderm of right limb bud is transplanted onto the mesenchyme of a left limb bud, the distal limb that develops will be that of a right limb with respect to muscle pattern and joint orientation (13, 14).
The PD axis seems to be determined by the length of time a mesodermal cell remains at the tip of the limb bud in the PZ under the influence of the AER. Once a cell leaves the tip, its position in the limb is fixed. Young tips grafted on older limb buds will duplicate existing limb elements, whereas older tips grafted on young buds will only form distal elements. The best hypothesis as to how this information is passed is that the number of rounds of cell division that occurs while under the influence of the AER determines the PD fate of a cell. Support for this hypothesis comes from experiments in which the limb bud is irradiated. The surviving cells of the irradiated tip have to undergo several extra rounds of mitosis before they can escape the influence of the AER and, thereby, gain positional determination. In these experiments, intermediate limb elements are not formed, just the preexisting proximal elements and newly formed distal elements (17).
Cellular differentiation of the homogenous, undifferentiated appearing mesenchymal cells in the limb bud results from different signals than those conveying the axis/positional information as described above. The center of the limb bud develops a condensation of cells that prefigures the skeletal elements, the chondrogenic core, which begins at the body wall and progresses distally with limb elongation. A rich vascular bed surrounds the chondrogenic core. Immediately adjacent to the vascular bed is a thick avascular zone that extends to the ectodermal sheath of the limb bud. Although the signaling mechanism has not been discovered, the ectoderm appears to control initial mesodermal differentiation by maintaining the adjacent mesenchymal cells in a flattened configuration, which prevents differentiation into chondrogenic cells. The central mesenchymal cells assume a rounded shape and form the chondrogenic core (16, 18). This process of differentiation occurs from proximal to distal. Early in the 7th week, cartilage anlage of the entire upper limb skeletal elements except the distal phalanges are present. Paddleshaped hand plates have formed by the end of the 6th week and condensations of cells have formed identifiable digital rays in the hand. The same is true of the foot 1 week later. The cells between the digital rays are a loose mesenchyme that undergoes programmed cell death (apoptosis) to create the separated fingers and toes.
After the chondrogenic anlagen of the future skeletal structures and the vascular bed develop comes the ingrowth of nerves, which is immediately followed by the development of muscle tissue. All bones are prefigured in mesenchyme, followed by cartilage, then bone. Actual bone appears toward the end of the embryonic period, first in the clavicle, mandible, and maxilla between the 6th and 7th weeks. Ossific centers appear soon after in the humerus, then radius, then femur, then tibia, and then ulna. Just prior to birth, ossific centers
appear in the calcaneus, talus, cuboid, distal femoral epiphysis, and proximal tibial epiphysis.
appear in the calcaneus, talus, cuboid, distal femoral epiphysis, and proximal tibial epiphysis.
The mechanisms controlling the development and patterning of the vasculature are not well worked out. Vascular cells are believed to have an intrinsic capacity to form vessels and branch that is controlled by inhibitory signals extrinsic to the angiotrophic tissues. Well-developed veins develop on the postaxial border of the limb buds and persist as the fibular and saphenous veins, permitting identification of the embryonic postaxial border even in mature organisms. The early preaxial veins, the cephalic and great saphenous veins, develop secondarily. The initial arterial supply to the limb bud organizes into a single axial artery. In the arm, this artery becomes the subclavian/axillary/brachial/anterior interosseous arteries. In the leg, the axial artery comes from the umbilical artery and becomes the inferior gluteal artery/sciatic artery/the proximal popliteal and the distal peroneal artery. The femoral and tibial arteries develop secondarily.
The brachial and lumbosacral plexuses and the major peripheral nerves are present by the 5th week. They progressively invade their target tissues and by the 7th week have innervated muscles and cutaneous tissues in the adult pattern. Each dermatome represents a single dorsal root’s sensory fibers. From cranial to caudal, the dermatomes of the limbs descend along the preaxial border and ascend along the postaxial border of the limb. Overlapping and variability amongst individuals make assessment of dermatomal sensation nonspecific for single nerves (Fig. 1-12).
Mesenchymal cells that are to become limb muscles migrate from the somatic layer of the lateral mesoderm during the 5th week and surround the chondrogenic core of the limb bud. They develop into dorsal and ventral groups from an undifferentiated mass and individual muscles gradually become distinct, again in a proximal/distal sequence. Most anatomically distinct adult muscles are identifiable in the 8th week. Mesenchymal cells develop into myoblasts which then elongate, form parallel bundles, and fuse into myotubes. Muscle-specific contractile proteins, actin and myosin, are synthesized and the myotubes form sarcomeres. By the 8th week, both myotube development and innervation are sufficiently advanced for movement to begin. By 12 weeks, the crossstriations of the myofibrils are apparent in myotube cytoplasm. Most muscle cells are formed prior to birth, with the remaining cells developing in the first year of life. Enlargement of muscles results from an increase in diameter with the creation of more myofilaments and elongation with the growth of the skeleton. Ultimate muscle size results from genetic programming, exercise, and the hormonal milieu.
Development of the synovial joints commences in the 6th week of development. A condensation of cells where the joint develops is called the interzone. The interzone cells differentiate into chondrogenic cells, synovial cells, and central cells. The chondrogenic cells are adjacent to the mesenchymal cells and form the articular cartilage. The central cells form the intra-articular structures. The synovial cells differentiate into both the tough fibrous capsule and the loose, vascular synovium. Programmed cell death (apoptosis) results in the cavitation that produces the joint per se. Motion is necessary for normal joint development as the host of conditions causing arthrogryposis demonstrate as well as animal experiments that create joint anomalies by paralyzing the developing fetus.
During the embryonic period, all four limbs are similar with parallel axes. The preaxial borders are cephalad and the postaxial borders are caudad. The thumb and hallux are preaxial; the radius/tibia and ulna/fibula are homologous bones occupying the same positions in the limb bud. The longitudinal axis at this stage passes through the long finger and
the second toe. During the fetal period, the upper limb rotates 90 degrees externally (laterally) and the lower limb rotates 90 degrees internally (medially). The forearm flexors come to lie medially and the forearm extensors laterally. The leg extensors lie ventrally and the leg flexors dorsally (Fig. 1-13).
the second toe. During the fetal period, the upper limb rotates 90 degrees externally (laterally) and the lower limb rotates 90 degrees internally (medially). The forearm flexors come to lie medially and the forearm extensors laterally. The leg extensors lie ventrally and the leg flexors dorsally (Fig. 1-13).
Thus, by the 8th week, the task of tissue differentiation is largely completed and growth is the major task ahead.
Molecular Insights of Limb Development.
The explosion in molecular biology and molecular genetic techniques has revealed much about how individual gene’s activation at specific moments in development causes the events that create complex organisms from single cells. The story is incomplete, and this section will highlight presently known or suspected molecular mechanisms that underlie development. The development of organs employs similar mechanisms of cell growth, differentiation, and patterning as occur in earlier development of the basic body plan. The mechanisms for differentiation and patterning are remarkably conserved from fruit flies to chicks to mice and to men.
The limb is one of the best studied body structures, and much information is available from the study of nonhuman animals, especially chicks, mice, and fruit flies. Much knowledge is inferential from the observations that certain genes and gene products are present at crucial moments in development. Often, many different genes and molecules are expressed simultaneously or in a closely overlapping sequence, and the complex interactions that control development are not fully worked out. The information presented in this section is based on the study of limb development in the chick except where noted. Most other information comes from gene “knockout” experiments in mice, wherein a specific gene is rendered nonfunctional and the effects on development are noted.
Limb Bud Outgrowth and Proximal-Distal Patterning.
As discussed previously, the AER is required for limb bud outgrowth (Fig. 1-14). The AER is a band of cells at the limb bud tip, lying between the dorsal and ventral limb ectoderm. Although the stimulus for AER formation, which resides in the mesoderm, is unknown, some of the molecular signals which are important
in specifying the location of the AER have been identified. Engrailed-1 (En-1) is a homeobox containing transcription factor whose expression is limited to the ventral limb ectoderm (19). Radical fringe (r-Fng) is a secreted factor that modulates signaling that is expressed only in the dorsal ectoderm (20). Radical fringe is a homolog of the Drosophila gene fringe, which helps specify DV boundaries in the fruit fly (21, 22).
in specifying the location of the AER have been identified. Engrailed-1 (En-1) is a homeobox containing transcription factor whose expression is limited to the ventral limb ectoderm (19). Radical fringe (r-Fng) is a secreted factor that modulates signaling that is expressed only in the dorsal ectoderm (20). Radical fringe is a homolog of the Drosophila gene fringe, which helps specify DV boundaries in the fruit fly (21, 22).
Excision of the AER results in truncation of the limb. The earlier the excision, the more proximal is the truncation. Limb bud outgrowth can be sustained after excision of the AER by insertion of beads carrying fibroblast growth factors. Fibroblast growth factors are a group of similar proteins that affect cell proliferation, differentiation, and motility. During development, they have in common a role in mediating mesenchymal-epithelial tissue interaction. To obtain the most normal limb development, two fibroblast growth factor (FGF)-soaked beads must be placed so that the polarizing region is mimicked as well as the AER (23). The absence of the mechanical flattening of the limb bud by the AER results in a bulbous limb bud and bunching of the digits. Nevertheless, fully differentiated limb skeletal structures can be produced.
FGF-2, -4, and -8 are expressed in the AER, and each is able by itself to sustain limb bud outgrowth (probably because of the ability of different FGFs to activate the same receptors) (24, 25 and 26). In vivo FGF-8 is found in the entire AER, while FGF-4 expression is limited to the posterior portion of the AER. FGF-10 and -8 are the critical FGFs expressed during the initiation of limb bud outgrowth. FGF-10 is expressed first in the lateral plate mesoderm at the site of the future limb bud. FGF-10 induces FGF-8 expression in ectodermal cells that will become the AER. Some experiments suggest that FGF-8 and -10 act in a positive feedback loop, that is, the expression of each supports and promotes the expression of the other. Mice in whom FGF-10 function is eliminated develop normally except for the complete absence of limbs and failure of normal pulmonary development (27).
Proximal-distal positional information is engraved upon individual cells in the PZ based on the length of time (number of mitoses?) the cell spends in the PZ as discussed earlier in this chapter (28, 29). Some experimental work suggests that transforming growth factor β (TGFβ) act in a gradient from the AER to increase cell adhesion by activating integrins— mediators of cell adhesion. Perhaps, the longer a cell is in the PZ, the more TGFβ it sees, and the more integrins are activated the greater the cell adhesion, and ultimately, the more distal is the limb positional information programmed into the cell.
Anterior-Posterior Axis Determination/Zone of Polarizing Activity (Fig. 1-15).
The ability of a small piece of tissue excised from the posterior and proximal limb bud to induce duplication of digits when grafted to an anterior position on another limb bud suggested that this region of polarizing activity synthesized a morphogen acting by a gradient to specify AP limb elements (15). If acting through a morphogenic gradient, the ZPA should give different digit patterns when transplanted to different areas of the limb bud, which it does (30). Furthermore, if a physical barrier is placed between the anterior and posterior parts of the limb bud, a normal number and order of digits is formed in the posterior portion of the limb bud, and no digits are formed anteriorly (31).
By serendipity, retinoic acid was found to cause reduplication of limbs in amputated salamanders (32). Subsequently, retinoic acid was identified in the limb bud with a high concentration posteriorly and a low concentration anteriorly. Retinoic acid on filter paper, placed anteriorly, induces mirror image digits to those formed from the natural posterior gradient (33). Nevertheless, retinoic acid is not a simple morphogen acting through a gradient. Bathing an entire limb bud in retinoic acid should eliminate the gradient, but instead mirror image reduplication occurs. Also, when retinoic acid concentration is at a minimum, the polarizing activity of the ZPA is
maximum. The action of retinoic acid is complex and includes several classes of nuclear retinoic acid receptors and retinoicbinding proteins in the cytoplasm. These different receptors and binding proteins are present in different amounts in various parts of the developing limb, suggesting a very complex role for retinoic acid. If retinoic acid synthesis is blocked, limb bud outgrowth does not occur or is severely stunted. At present, it appears that retinoic acid acts by regulating the cells that can express a protein called sonic hedgehog (34). It is likely that a Hox gene is an intermediate that is stimulated by retinoic acid and the Hox gene expression specifies the sonic hedgehogexpressing cells. If retinoic acid receptors are blocked after sonic hedgehog expression has been established, limb malformations still occur, suggesting some additional role in limb patterning, perhaps through its affect on Hox genes (34). Retinoic acid is interesting and important, not only for its role in normal development, but because it is a powerful teratogen. Various retinoids, which are derivatives of vitamin A, have been used in pharmacological doses mainly to treat dermatologic conditions such as types of acne, psoriasis, exfoliative dermatitis, and disorders of keratinization. The retinoids have been associated in animal and humans with multiple different birth defects, and the systemic usage of retinoids is not recommended for 2 years after discontinuing systemic retinoids, because of the prolonged elimination half-life of these compounds (35).
maximum. The action of retinoic acid is complex and includes several classes of nuclear retinoic acid receptors and retinoicbinding proteins in the cytoplasm. These different receptors and binding proteins are present in different amounts in various parts of the developing limb, suggesting a very complex role for retinoic acid. If retinoic acid synthesis is blocked, limb bud outgrowth does not occur or is severely stunted. At present, it appears that retinoic acid acts by regulating the cells that can express a protein called sonic hedgehog (34). It is likely that a Hox gene is an intermediate that is stimulated by retinoic acid and the Hox gene expression specifies the sonic hedgehogexpressing cells. If retinoic acid receptors are blocked after sonic hedgehog expression has been established, limb malformations still occur, suggesting some additional role in limb patterning, perhaps through its affect on Hox genes (34). Retinoic acid is interesting and important, not only for its role in normal development, but because it is a powerful teratogen. Various retinoids, which are derivatives of vitamin A, have been used in pharmacological doses mainly to treat dermatologic conditions such as types of acne, psoriasis, exfoliative dermatitis, and disorders of keratinization. The retinoids have been associated in animal and humans with multiple different birth defects, and the systemic usage of retinoids is not recommended for 2 years after discontinuing systemic retinoids, because of the prolonged elimination half-life of these compounds (35).
Liver and central nervous system toxicity, as well as anemia and hyperlipidemia, may occur with systemic retinoid use. These problems usually resolve with the discontinuation of retinoid therapy. Rheumatologic complications of retinoid use include hyperostosis, arthritis, myopathy, vasculitis, and a condition mimicking seronegative spondyloarthropathy (36).
The sonic hedgehog gene is expressed in the ZPA. Activation of this gene, through cell transfection, in anomalous locations in the limb bud will cause digit duplication in the same manner as transplantation of the ZPA tissue (37). It appears that sonic hedgehog stimulates the gene for BMP-2 (38). The gene activation of both occurs in the same cells with sonic hedgehog preceding that of BMP-2. The bone morphogenic proteins are members of the TGFβ superfamily and BMP-2 is, specifically, a homolog of a fruit fly gene that specifies segment polarity—making it a good candidate for an axis determining gene. BMP-2 is secreted from cells and, therefore, its action extends over a larger area than just the cells in which it is produced. BMP-4 expression overlaps that of BMP-2, and it is probably involved in AP axis specification as well. Sonic hedgehog in the mesenchyme also appears to participate in a positive feedback loop with FGF-4 in the ectoderm which may be important in maintaining the AER and supporting continued limb outgrowth and patterning (39). BMP-2 and BMP-4 also function in regulating the size and shape of long bones. Overexpression of these genes appears to cause and increase in the quantity of mesenchymal cells that differentiate into the chondrogenic precursors of the skeleton.
It appears that BMP-2 and BMP-4 are involved, as well, in the molecular mechanisms of joint formation. If noggin, a BMP inhibitor, is not present, joints do not form. Present evidence suggests that members of the Hox A and Hox D families are regulators of BMP and GDF-5 (growth and differentiation factor-5). GDF-5 (a BMP-related protein) is expressed specifically in the prospective joint region. If noggin is not present, GDF-5 is not expressed, and BMP-2 and BMP-4 are not inhibited resulting in a continuous, jointless skeleton. A balance of activating and inhibiting signals seems to be necessary for normal joint cavitation (40, 41).
Some experimental work suggests that cell-extra cellular matrix interactions are involved in the mechanism of joint cavitation. Specifically, CD44s, an isoform of a cell surface receptor that interacts with hyaluronan, is found in a single layer of cells outlying the presumptive joint cavity in rats (42). One hypothesis is that the creation of a hyaluronanrich, but proteoglycan- and collagen II-poor, extracellular matrix results in a loss of cell adhesion and allows joint cavity formation (42).
Dorsal-Ventral Axis Patterning/Ectodermally Controlled Patterning.
Experiments in which limb bud ectoderm is transplanted onto mesoderm with reversal of its DV axis reveal that digits, muscles, and tendons conform to the axis of the overlying ectoderm (13). Several genes involved in DV specification have been identified. Evidence suggests that Wnt-7A, a secreted signaling protein, confers the dorsal character to the ectoderm and it stimulates Lmx-1 (43). Lmx-1 is a homeobox gene that encodes a transcription factor that dorsalizes mesoderm. The ventral limb expresses the homeobox-containing transcription factor engrailed (En-1). En-1 appears to suppress Wnt-7A expression, thereby limiting the activity of Lmx-1 to the dorsal mesenchyme (44).
The actions of several genes are important in determining more than a single axis. For example, Wnt-7A expression is necessary for normal AP digit formation and sonic hedgehog is necessary for limb outgrowth, proximal-distally, in addition to AP patterning. The coordinated development of all three axes is believed to be regulated by interactions of several signaling genes. Wnt-7A, sonic hedgehog, and FGF-4 are promising candidates for this role (45, 46).
Both the AER and the ZPA activate HOX A and HOX D genes. HOX genes are the vertebrate homologs of the fruit fly homeogenes which specify body segment identity. HOX D genes are expressed in an overlapping, nested fashion in the AP axis of the developing limb (47) (Fig. 1-16). HOX D gene can also be activated by sonic hedgehog alone. HOX A genes have an overlapping expression along the dorsal axis, suggesting a role in specifying limb components. HOX genes have specifically been shown to affect the growth of cartilage and precartilaginous condensations (48, 49). They may be important in determining the length, segmentation, and branching of limb elements. HOX genes are not expressed strictly along AP or DV axes, and their position of expression differs in various areas of the limb. Human limb malformations caused by mutations in Hox genes have been identified and are discussed in the section on clinical correlates. Hox genes’ activity overlaps and is sufficiently redundant that a mutation of a single Hox gene generally results in a minor limb anomaly.
The specific signals and mechanisms that control muscle, ligament, and tendon development have not been identified. Exploration of these mechanisms may ultimately be of great clinical utility.
Clinical Significance.
Limbs are extremely susceptible to anomalies, probably due to their complex developmental biology and their exposed position outside of the body wall. Nearly all teratogens and chromosomal anomalies have ill effects on limb development. A large number of single gene mutations disturb the normal development of limbs as well. Rapid advances in molecular genetics are resulting in the identification of the gene(s) responsible for many Mendelian disorders. Developmental molecular biologists are identifying genes that create limb deformities in animals, whose homologous human genes may be responsible for other specific limb defects.
Limb deficiencies occur in 3 to 8 per 1000 live births (50). One-half of limb deficiencies occur as isolated defects and the other half occur with associated malformations (50). These associated malformations may be life threatening. The most common associated malformations are musculoskeletal, head and neck, cardiovascular, gastrointestinal, and genitourinary.
As discussed previously, destruction of the AER, the PZ, or failure of expression of critical signaling molecules can result in truncation or absence of limb development. Although specific defects have not been identified in humans, there are several inherited disorders with limb deficiencies that may well have a mutation in a specific signaling molecule. These include Roberts syndrome with phocomelia; Acheiropodia (Brazil Type) with absent hands and feet; “Buttiens: distal limb deficiencies syndrome”; and CHILD syndrome with variable transverse and longitudinal deficiencies. A mouse mutant with a single digit has been found to have a mutation in the Hoxa-13 gene (51). Human split hand/split foot deformity (lobster claw deformity) is characterized by a failure of central ray formation, and at least one causative gene has been localized, but not yet identified (52).
Vascular anomalies or accidents probably cause some limb deficiencies by prohibiting limb outgrowth or by causing necrosis of already differentiated limbs (53, 54). Amniotic bands result in amputation by interfering with vascular supply (50).
Intercalary limb deficiencies, with absence of proximal structures, may result from a temporary injury to the PZ as discussed in the section Developmental Anatomy of the Limb.
Synostoses are failures of joint formation or separation of adjacent bone, such as the radius and ulna, with a single exception. That exception is distal syndactyly caused by amniotic bands (55). The vast majority of synostoses are inherited, but only a few of the mutated genes have been identified. Identification of the causative genes is likely in the near future and will shed much light on the mechanisms of normal joint formation and of normal bone modeling from the mass of undifferentiated mesenchymal cells that prefigure the skeletal elements. Nearly 100 disorders have synostosis as a feature. The mouse mutant limb deformity gives a flavor for the kind of causation of these deformities. Limb deformity mice are characterized by fusion of adjacent bones such as the radius and ulna. The limb bud is narrow and the AER is patchy (56). A decrease in sonic hedgehog expression and an absence of FGF-4 expression has been found (57).
Teratogens such as thalidomide and alcohol can cause synostoses in humans. Retinoic acid creates synostoses in animals when applied during chondrogenesis of developing limbs (58).
Failure of programmed cell death may be an important factor in causing some disorders with synostosis as a feature. A failure of aphotic cell death in the interdigital mesenchyme is presumed to be the cause of some syndactyly.
Excessive partitioning of skeletal elements are usually inherited conditions. Triphalangeal thumb is the most common of these disorders. Several affected families’ abnormality has been linked to a region on chromosome 7, but the gene has not been identified (59, 60). Other families do not link to this locus, implying that more than one gene mutation causes triphalangeal thumb.
Polydactyly, whether isolated or associated with other anomalies, is usually an inherited condition. Given the complex interactions that occur in limb pattern specification, it is not surprising that a number of causes of this condition are being found.
Grieg cephalopolysyndactyly is an autosomal dominant disorder characterized by postaxial polysyndactyly of the hands and preaxial polysyndactyly of the feet and dysmorphic facies.
A DNA-binding transcription factor, named GLI 3, is the cause of this disorder (61). GLI 3 expression is restricted to the interdigital mesenchyme and joint-forming regions of the digits. A mouse mutant with a defect in the homologous gene has ectopic expression of both sonic hedgehog and FGF-4 in the anterior limb bud (62). Another mutation causing human polydactyly is in the Hox D cluster of homeobox genes that has been implicated in digit specification. Synpolydactyly is caused by a mutation of Hox D-13 (63). Smith-Lemli-Opitz syndrome is characterized by a variety of birth defects including postaxial polydactyly and brachydactyly. Cholesterol synthesis is defective in children with this syndrome and sonic hedgehop utilizes cholesterol as a transport molecule. It is possible that the limb anomalies in this syndrome result from a distortion of the normal sonic hedgehog gradient due to cholesterol insufficiency.
A DNA-binding transcription factor, named GLI 3, is the cause of this disorder (61). GLI 3 expression is restricted to the interdigital mesenchyme and joint-forming regions of the digits. A mouse mutant with a defect in the homologous gene has ectopic expression of both sonic hedgehog and FGF-4 in the anterior limb bud (62). Another mutation causing human polydactyly is in the Hox D cluster of homeobox genes that has been implicated in digit specification. Synpolydactyly is caused by a mutation of Hox D-13 (63). Smith-Lemli-Opitz syndrome is characterized by a variety of birth defects including postaxial polydactyly and brachydactyly. Cholesterol synthesis is defective in children with this syndrome and sonic hedgehop utilizes cholesterol as a transport molecule. It is possible that the limb anomalies in this syndrome result from a distortion of the normal sonic hedgehog gradient due to cholesterol insufficiency.
Skeletal dysplasias are a heterogeneous group of disorders whose genetic causes are rapidly being discovered, giving insight into the mechanisms of normal and disordered skeletal development (see Chapter 7).
Many single and groups of congenital anomalies that occur sporadically are believed to result from vascular disturbances in the embryo or fetus. The best developed of the vascular causation hypotheses is the subclavian artery disruption sequence, which seeks to explain Klippel-Feil syndrome, Poland anomaly, Möbius syndrome, absence of the pectoralis major, terminal transverse limb deficiencies, and Sprengel deformity. A disruption occurs when a normal embryo suffers a destructive process with cascading consequences. Since all the tissues affected in these various disorders receive their major blood supply from the subclavian artery, it is hypothesized that a defect of arterial formation or an injury to existing arteries causes these defects. The location and extent of tissue abnormality is determined by the extent, location, and timing of the interruption of normal blood supply. The observation underlying this hypothesis is that the disorders listed above often occur together in various combinations.
Possible mechanisms resulting in arterial ischemia include vessel occlusion from edema, thrombus, or embolus; extrinsic vessel compression due to surrounding tissue edema, hemorrhage, cervical ribs, aberrant muscles, amniotic bands, or uterine compression; abnormal embryologic events, including delayed or abnormal vessel formation and disruption of newly formed vessels; and environmental factors such as infection, hyperthermia, hypoxia, vasculitis, or drug effects. It is possible that some fetuses suffer ischemia due to normal embryologic events that are idiosyncratically not well tolerated, such as the rapid descent of the heart and great vessels.
The vascular accident hypothesis is attractive in explaining combinations of congenital anomalies and their, usually, sporadic occurrence. However, there are often combinations of anomalies that are difficult to relate to a single vascular event and the question of whether the anomalies resulted in, rather than being caused by, vascular abnormalities is not easily resolved.
Amniotic bands or constriction rings cause a large portion of nonhereditary congenital limb anomalies. Constriction rings are diagnosed by the occurrence of soft-tissue depression encircling a limb or injured from amputations or disruptions. Constriction rings are commonly multiple and may be broad or narrow. The depth of the ring determines whether the limb distal to the ring is normal, hypoplastic, engorged (from venous or lymphatic obstruction), or amputated (from vascular insufficiency). Syndactyly, clubfoot, and clubhand have also been associated with constriction rings.
The origin of constriction rings is uncertain. The mechanisms by which amniotic strands might form are disputed (64, 65, 66 and 67). One hypothesis holds that amnion adheres to areas of preoccurring hemorrhage and by itself is not pathogenic (68). Nonetheless, the syndrome is common, affecting 1 in 5000 to 15,000 births. Recurrence risks of this syndrome are low (50).
DEVELOPMENTAL ANATOMY OF THE VERTEBRAL COLUMN
The vertebral column, be it cartilage or bone, defines the species of the subphylum of “vertebrates.” Evolution of a vertebral column to replace the notochord allows a strength, flexibility, and protection to the neural tube that conferred many advantages to vertebrate species. Minor and even major anomalies of the vertebral column are compatible with life and good function. Vertebral column development depends on the appropriate prior development of the notochord and somites.
While the mesoderm is forming during gastrulation, a mass of ectodermal cells proliferates and forms the archenteron—a tube that migrates cranially in the midline between the ectoderm and the endoderm. The floor of the archenteron forms the notochordal plate. For a short time, there is a direct connection between the primitive gut and the amniotic cavity since the endodermal floor is not continuous and the blastopore (the opening of the archenteron) communicates with the amniotic cavity. This connection is obliterated by the end of the 3rd week, and remnants of this connection are presumed to be responsible for diastematomyelia.
The notochord arises from cells in the primitive streak which come from the ingress of cells from the epiblast during gastrulation and, later, from the caudal eminence. This ingress of cells forms the endoderm as well as the notochord and the paraxial mesoderm (segmental plate). The notochord develops from cranial to caudal by adding cells as it develops. It is initially a solid rod in which a small central canal develops. The notochord induces the formation of the neural groove, which gradually closes to form a tube with a central canal. By the 23rd day, the neural groove is closed except at its most cranial and caudal ends. These openings are termed the neuropores, which close by the end of the 4th week. Closure of the neural tube progresses from cranially to caudally. Failure to close properly is hypothesized to be the cause of NTDs such as myelomeningocele.
The somites also develop from cells that are internalized through the primitive streak. These cells form the paraxial
mesoderm that will become the somites and ultimately become the vertebrae. Pre-somitic cells cluster by increased adhesion into distinct balls of epithelial cells surrounding mesenchymal cell giving the embryo its first segmental organization. Positional information is programmed into the somites by the time they are morphologically distinct. For example, a thoracic somite will still form a rib when transplanted into the cervical region. The positional information is imparted during gastrulation (69, 70). Somites do not depend on interaction with the neural tube or notochord for development and fated cells will develop into somites in vitro.
mesoderm that will become the somites and ultimately become the vertebrae. Pre-somitic cells cluster by increased adhesion into distinct balls of epithelial cells surrounding mesenchymal cell giving the embryo its first segmental organization. Positional information is programmed into the somites by the time they are morphologically distinct. For example, a thoracic somite will still form a rib when transplanted into the cervical region. The positional information is imparted during gastrulation (69, 70). Somites do not depend on interaction with the neural tube or notochord for development and fated cells will develop into somites in vitro.
Four occipital, 8 cervical, 12 thoracic, 5 lumbar, 5 sacral, and 4 or 5 coccygeal pairs of somites will develop. The first somites are evident at three and a half weeks and 30 pairs are present at four and a half weeks. Not all of the somites are visible simultaneously. Somites develop a complex internal organization. The somite begins as a ball of pseudostratified epithelium surrounding a central cavity, the somitocoel. The central cavity becomes filled with mesenchymal cells. Some of these cells, along with cells in the medioventral portion of the somite become the sclerotome. Cell from the sclerotome will form the vertebral bodies and vertebral arches and emerge without the epithelial portion of the sclerotome to surround the neural tube. In addition to contributing to the sclerotome, cells from the central cavity migrate to become the intervertebral discs and contribute to rib formation. The dorsolateral wall of the somite is called the dermomyotome. It separates into the dermatome laterally and the myotome lying between the dermatome and the sclerotome. The dermatome gives rise to the dermis of the skin and the myotome supplies cells for muscle, tendons, and fascia (Fig. 1-17).
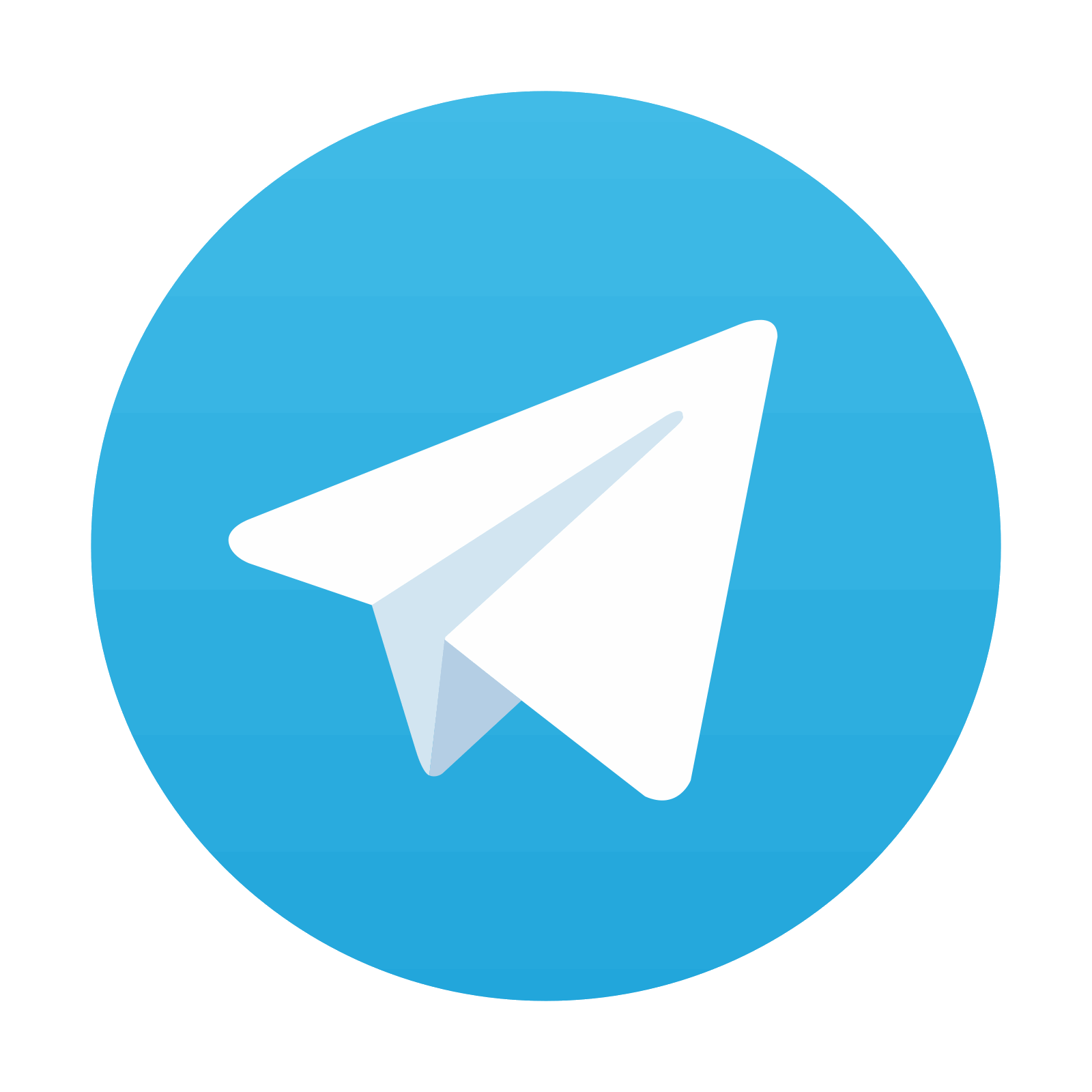
Stay updated, free articles. Join our Telegram channel
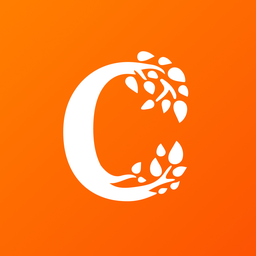
Full access? Get Clinical Tree
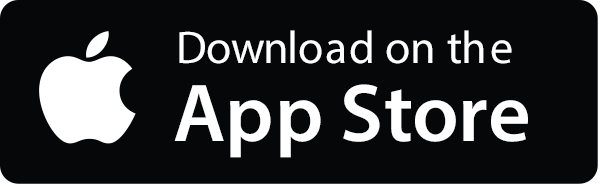
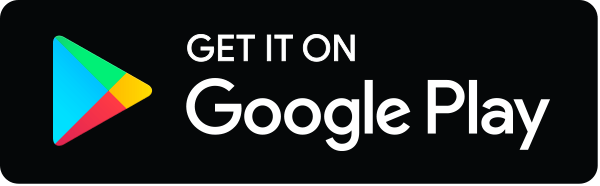