7 How might a non-progressive central nervous system lesion lead to progressive musculoskeletal deformity and impairment of function? How early does muscle deformity occur in children with cerebral palsy (CP)? Is it possible that intervention in infancy and early childhood may alter the development of muscle deformity? Although we lack empirical evidence, our growing understanding of normal skeletal muscle development and growth suggests that muscle deformity in CP may be related to an impairment of growth and to subsequent adaptation to altered use. To explore this concept and its implications, it may be helpful to first review normal skeletal muscle structure and function and the alterations described in muscle in CP, and then discuss normal skeletal muscle development and growth. We can then consider how early muscle development may be altered in children with CP, how skeletal muscles may respond to subsequent use in children with CP, and the implications this may have for intervention. Muscle structure and function, and the alterations noted in children with CP, have been discussed in detail in Chapter 6, and the development of the corticospinal tract and spinal cord networks have been discussed in Chapter 2. They are discussed briefly again below to facilitate discussion of the questions raised above. Skeletal muscle contraction occurs through the interaction of two proteins, actin and myosin. These proteins form filaments that overlap in the sarcomere, the basic functional unit of the muscle cell. In the presence of calcium, actin binds to myosin and the resulting configurational change in myosin results in a relative change in the position of the actin and myosin filaments. A range of structural proteins are needed to facilitate and stabilize this interaction. Other intracellular proteins form supportive structures called costameres which transmit the force generated by the interaction of the actin and myosin filaments through the cell membrane. The costameres link the sarcomeres to the basal lamina, which is in turn linked to a continuous three-dimensional network of connective tissue surrounding fibres, fascicles and whole muscles which is connected to internal and external tendons and to the surrounding fascia (for a review see Purslow, 2002). Human muscles may be classified depending on the predominant type of myosin heavy chain (MHC) isoform present into slow (MHCIβ) and fast (MHCIIa, MHCIIx) muscles, although rather than having discrete muscle fibre types each muscle fibre may contain a range of different MHC isoforms (Pette and Staron, 2000). Thyroid hormone up-regulates the genes expressing fast MHC isoforms, whereas nerve activity-dependent transcription of the genes expressing MHCIβ is necessary for the development and maintenance of slow fibres (Schiaffino et al., 2007). The MHC isoform expressed by a muscle cell varies depending on how the muscle is used: there is a shift towards a slower MHC isoform with more frequent and tonic muscle activation, whereas with reduced or phasic activity there is a shift towards a faster MHC isoform (Pette and Staron, 2000; Schiaffino et al., 2007). Skeletal muscle is a highly adaptable tissue, and is able to respond to altered demand by changes in the expression of genes within the cell nucleus. Factors influencing muscle gene expression include the pattern of neuronal activation, the energy substrates available and the presence of local hormones and growth factors (Fig. 7.1). Signalling pathways allow a balance between active muscle protein synthesis and active protein degradation, which in turn determines the size and function of the muscle cell (Gundersen, 2011). Innervation, growth factors and nutrition appear to promote protein synthesis, while denervation, immobilization, systemic inflammation and starvation result in active protein degradation and muscle atrophy (Gundersen, 2011) (Fig. 7.2). Growth factors may have different actions on different components of the muscle: myostatin, which inhibits muscle fibre growth, is important in muscle repair and appears to promote growth of the connective tissue matrix (Zhu et al., 2007). Insulin-like growth factor 1 (IGF-1) is released by the muscle when active and promotes protein synthesis within the muscle fibre through pathways involving mammalian target of rapamycin (mTOR) (Clemmons, 2009; Otto and Patel, 2010). Protein synthesis in muscle may also be stimulated directly by ingested essential amino acids, particularly leucine, acting through mTOR (Drummond et al., 2009). This action of ingested amino acids may, however, be inhibited during periods of starvation or stress (Fujita et al., 2007). The mitochondrial content of the sarcomere, and the ability of the sarcomere to use energy substrates such as glucose or fat, appears to be influenced by pathways within the cell that are sensitive to neuronal activation such as those involving the calcineurin-NFAT (nuclear activated factor of T cells) system (Schiaffino et al., 2007; Westerblad et al., 2010). Calcineurin-NFAT also appears to be important in determining the type of MHC expressed, which will influence the contraction speed of the muscle (Pette and Staron, 2000; Schiaffino et al., 2007). Skeletal muscle responds to both increased use and decreased use: cast immobilization, for instance, will lead to a reduction in muscle fibre protein synthesis and a shift towards protein degradation within the muscle fibre. This is an active process that involves the tagging of muscle fibres by ubiquitin prior to proteolysis and in which myostatin appears to be involved (Gundersen, 2011). Cast immobilization of the quadriceps in the human results in a reduction in protein synthesis within 48 hours (Urso et al., 2006) and also in a reduction of the normal protein synthesis response of muscle to ingested amino acids (Glover et al., 2008). A muscle fibre thus consists of a composite and complex network with a close balance and interaction between contractile and non-contractile elements which is influenced by a number of factors, including innervation, the mechanical load imposed, and the presence of local or systemic growth factors. Of these factors, the most important factor appears to be the pattern of neuronal activation and the ionic changes and mechanical stresses associated with contraction. A focal impairment of muscle growth with replacement of the muscle fibres by fat and fibrous tissue was noted in neonatal mouse models of brachial plexus palsy following neurotomy (Kim et al., 2010; Nikolau et al., 2011) or denervation using botulinum toxin A (Kim et al., 2009). Neuromuscular electrical stimulation has been shown to lessen reductions in muscle volume, and to moderate the increase in fat content and the reduction in muscle oxidative capacity after chronic spinal cord injury in humans (Biering-Sorenson et al., 2009). Muscle protein synthesis is suppressed during periods of active contraction, but there appears to be a subsequent increase in protein synthesis (Atherton and Rennie, 2009). Stretch without contraction in the human gastrocnemius does not appear to lead to protein synthesis (Fowles et al., 2000), and cultured muscle cells show a prolonged inhibition of protein synthesis after mechanical stretch without activation (Atherton et al., 2009). Rather than resulting in muscle growth, repeated passive stretch instead appears to promote expression of the atrophy pathway in the rat soleus (Gomes et al., 2006). Van Dyke et al. (2012) recently showed that passive stretch of the adult rat soleus following tenotomy did not alter the rate of sarcomere loss in comparison to unstretched tenotomized muscle: sarcomere loss was reduced but not prevented when stretch was combined with electrical stimulation of the muscle. Each skeletal muscle fibre is innervated by an alpha-motor neuron (αMN): the αMN and its associated muscle fibres form a motor unit (MU). There is a close relationship between the size of a MU, the size of the αMN, and the force produced by the MU. Small MUs have a small number of slow fibres innervated by a small αMN, and large MUs have a large number of fast fibres innervated by a large αMN. These MUs are distributed throughout the muscle and allow the graded development of the force of contraction (Henneman and Olson, 1965); small motor units are activated initially, and as the force required increases, larger motor units are recruited and the rate of contraction of individual motor units is increased. This allows smaller fibres with greater endurance to be used more often, and reserves the energy-expensive large MUs for movements where speed and force of contraction are important. Muscles are composed of multiple motor units distributed over large volumes of the muscle: the combination of a mechanically robust connective tissue framework and a distributed MU network allows the smooth transmission of lateral and axial forces. There is, as yet, limited information on skeletal muscle changes in children with CP. Muscle biopsy studies in this group have noted marked fibre size variability and predominance of either fast or slow fibres: this may reflect the heterogeneous functional level of the children involved and variation in the muscles assessed. In a recent systematic review, Barrett and Lichtwark (2010) noted that the most consistent morphological and structural change evident in the muscles of children with spastic CP was reduced size, as indicated by reduced muscle volume, reduced cross-sectional area and reduced muscle thickness. There have been conflicting reports on muscle fibre length changes, with some groups reporting no alteration in muscle fibre lengths and other groups reporting reduced muscle fibre lengths. Most of the studies on muscle fibre lengths involved the gastrocnemius muscle: recently, Moreau et al. (2009) described muscle fibre shortness in the rectus femoris and unchanged muscle fibre lengths in the vastus lateralis, suggesting that rather than a uniform change, the alteration seen in muscle in children with CP may reflect a combination of influences, including the nature of the central lesion, the level of function of the child, the morphology of individual muscles, and possibly the effect of previous intervention. Lieber et al. (2004) have assessed the morphology of the flexor carpi ulnaris in children undergoing surgery and noted increased sarcomere lengths and increased stiffness of muscle fibres: whether these changes are primary or secondary is unclear. In a recent study, this group assessed biopsies from the semitendinosus and gracilis muscles in 17 cases with CP and 14 controls (Smith et al., 2011). They found markedly reduced muscle fibre diameter and reduced muscle cross-sectional area. Sarcomere lengths appeared to be increased at rest compared to controls: this was more marked with increasing deformity and with greater limitation of function. The involved muscles were also stiffer due to increased collagen content. Muscle function appears to be altered in children with CP. Stackhouse et al. (2005) investigated the quadriceps femoris and triceps surae muscles in children with CP: they found that children with CP had reduced muscle strength due to impaired activation of the muscles and to co-contraction of antagonist muscles. Voluntary activation of the quadriceps was reduced by 33% and 45% in triceps surae in the CP group in comparison to controls, suggesting a limitation in the extent to which muscle fibres could be activated in response to increasing demand in children with CP. There may also be limitation in rate-coding, which is the ability of a muscle to increase the strength of contraction by increasing the frequency of contraction. Rose and McGill (2005) looked at voluntary activation of the gastrocnemius and tibialis anterior in children with CP. They found a similar activation rate to controls at lower frequencies of activation but noted that the children with CP were unable to increase the frequency of activation of smaller MUs, and as noted by Stackhouse et al., (2005), were unable to recruit larger (higher threshold) MUs. The speed of contraction and the speed of relaxation may also be altered: Downing et al. (2009) looked at the rate at which a moment could be generated and relaxed across the lower limb joints of ambulant children with CP. They found that children with CP took 89% longer to generate a peak moment and 71% longer to reduce the moment in comparison to controls: this was particularly marked in the distal muscles of the lower limb and was felt by the authors to be of sufficient severity to adversely affect ambulation. This may be related to altered or prolonged activation of the αMN by the spinal cord networks or to changes of oxidative metabolic capacity or calcium control within the muscle cell, as noted by Smith et al. (2009). The changes seen in gross muscle function and morphology in CP are mirrored by changes in gene activation and expression within the sarcomere. Smith et al. (2009) looked at the pattern of gene expression in the flexor carpi ulnaris of children with CP and noted a shift towards a faster muscle phenotype with expression of MHCs not usually seen in children. These included the fetal and perinatal isoform and even MHCIIb, which is normally not expressed in humans, despite up-regulation of the pathway for slow oxidative fibre-type determination. They also noted increased levels of parvalbumin, a calcium-binding protein which would affect the relaxation rate. They noted that their findings did not fit a simple model of increased use or disuse of muscle. Ponten et al. (2008) looked at MHC expression in the flexor carpi ulnaris in children with CP with different levels of functional involvement: she found a shift towards greater expression of MHCI in children with greater levels of function and of MHCII isoforms in children with reduced upper limb function. The timing of onset of muscle deformity in children with CP is not clear. A reduction in medial gastrocnemius volume, PCSA and fascicle length has been noted in children with spastic CP aged between 2 and 5 years associated with a reduction in passive dorsiflexion (Barber et al., 2011), suggesting that muscle changes may occur at an earlier age than is generally considered. Before discussing how this may occur, it may be helpful to briefly review our understanding of normal skeletal muscle development and growth. Primary myotubes are formed in the human embryo at around eight post-conception weeks (PCW) following migration of myoblasts into the developing limb bud (Ijkema-Paassen and Gramsbergen, 2005). Activation of muscle fibres by αMNs occurs soon after formation of the myotubes, and muscle contraction is seen between eight and ten PCW (Prechtl, 1993). This muscle contraction occurs before ingrowth of the corticospinal tract (CST), and appears to represent the activity of intrinsic spinal cord networks capable of ordered discharge without corticospinal or afferent input (Vinay et al., 2002). The afferent (sensory) axons from skeletal muscle appear to reach the spinal cord grey matter by 7.5 PCW and make dense connections (which are later refined) to innervate motor neurons by 8.5–9 PCW, going on to establish the monosynaptic reflex pathway by 14 PCW (for a review, see Clowry, 2007). Muscle fibres in the adult human are each innervated by a single axon but initially each muscle fibre is innervated by a number of αMNs: this is termed polyneuronal innervation. Regression of polyneuronal innervation occurs in the rat soleus prior to the onset of locomotion (Ijkema-Paassen and Gramsbergen, 2005), but there is limited evidence about the timing of neuronal regression and the development of mononeuronal activation in the human. Gramsbergen et al. (1997) studied the innervation of human psoas fibres and found that mononeuronal activation occurs by 12 weeks postnatally. Development of motor function appears to be greatly dependent on the ingrowth of the CST to the spinal cord (see Fig. 7.3). The CST appears to have a number of functions including control of afferent inputs, control of spinal reflexes, direct and indirect excitation of motor neurons, and inhibition of motor neurons, together with trophic effects on spinal networks during development (Lemon and Griffiths, 2005). CST axons invade the human spinal cord from 17 to 29PCW and innervate the ventral horn from 31 to 35PCW, influencing the development of the cord networks, in particular the development of inhibitory interneurons (Clowry, 2007). Martin et al. (2005) identified three CST developmental stages: namely, growth of axons to the cord; refinement of grey matter termination; and development of motor control. He suggested that refinement and elimination of cord synapses occur between 1 and 2 years of age in humans and may be related to activity-dependent competition between developing CST terminals and other spinal neural systems. As the corticospinal system and cord networks develop, the motor cortex can activate cord circuits and αMNs with lower levels of input through the use of postsynaptic facilitation, increased neurotransmitter expression, and an increase in CST axon branches (Chakrabarty and Martin, 2010). Regression of polyneuronal innervation of muscle appears to occur after the onset of CST innervation (Clowry, 2007) and is likely to be influenced by the effect of the CST on cord networks. The refinement of spinal cord networks appears to be important for αMN function. Eken et al. (2008) studied MU activity in rat soleus muscles and found that the mean duration of muscle activity increased from 3.4 seconds at seven days to 62 seconds in adults, with most of this increase occurring after the age of three weeks. They suggested that postnatal development of tonic firing in soleus MUs depends on concurrent appearance of monoamine-dependent plateau potentials in αMNs due to interaction between the developing CST and intrinsic cord networks. The neuromuscular junction also undergoes development during this period. In the rat neuromuscular junction, a gradual change from immature, low-threshold calcium channels associated with the acetylcholine receptor to faster, higher threshold channels in the mature muscle has been noted (Navarrete and Vrbova, 1993). These immature channels may be re-expressed following denervation (Hughes et al., 2006). The refinement of intrinsic cord networks due to interaction with the CST thus appears to allow amplification of the CST input, development of tonic activation of muscle, maturation of the neuromuscular junction, regression of polyneuronal innervation and the refinement of afferent input (Chakrabarty and Martin, 2010; Clowry, 2007; Eken et al., 2008; Martin, 2005). Primary myotubes express embryonic MHC and the form of MHC seen in adult slow muscle, MHCIβ (Biressi et al., 2007). Secondary myotubes, formed by a subsequent wave of migrating myoblasts, express embryonic and perinatal MHC isoforms. Although MHC expression in primary myotubes appears independent of innervation, the development of subsequent muscle fibres and their expression of MHC isoforms appears to be closely related to their pattern of innervation (Ijkema-Paassen and Gramsbergen, 2005). There is limited data on MHC expression in the human fetus. Schloon et al. (1979) noted a predominance (95%) of fast fibres before 34 weeks’ gestation, with an increase in the percentage of slow fibres to 40% at term, but the histochemical classification used would have included developmental MHC isoforms (embryonic and perinatal) as well as MHCII muscle fibres. Studies in mice (Agbulut et al., 2003) suggest a sequential expression of MHC isoforms due to down-regulation of developmental (embryonic and perinatal) isoforms, and up-regulation and stabilization of the adult MHC isoforms, with MHCIIa and MHCIIx expressed postnatally. Muscle fibre growth and the development of the connective tissue network of muscle appears to be marked in the antenatal and perinatal period. In the human, the muscle fibres in sartorius appear to double in diameter between midgestation and term (Moore et al., 1971) and an accelerated growth in overall muscle fibre diameter in humans has been noted between 35 weeks’ gestation and term (Schloon et al., 1979). This early marked growth of muscle fibres appears to be dependent on an adequate supply of protein in the form of amino acids: this has been noted to be most marked in pigs in late gestation and in the perinatal period (Brameld et al., 1998). Muscle protein synthesis in neonatal pigs is associated with an increased sensitivity to growth hormones, insulin and amino acids: this increased sensitivity appears to decrease with age (Suryawan et al., 2007) and in the neonatal pig is associated with a marked increase in individual mitochondrial activity associated with an increase in mitochondrial number (Schmidt and Herpin, 1997). The importance of early nutrition in muscle growth is also suggested by studies on the effect of the timing of nutritional restriction in animal studies. In rats, under-nutrition prior to weaning has been shown to cause permanent stunting of muscle growth. In contrast, the effect of under-nutrition subsequent to weaning can generally be reversed (Bedi et al., 1982). Skeletal muscle is likely to be an expensive tissue in terms of protein requirements, and muscle growth may be selectively limited in the antenatal or perinatal period if nutrition is impaired. A relative inhibition of fetal skeletal muscle growth has been noted in a sheep model of late placental insufficiency/intrauterine growth retardation, suggesting that skeletal muscle growth may be preferentially down-regulated if early nutrition is compromised (Thorn et al., 2009). Because of the pennate nature of skeletal muscle, skeletal muscle growth involves changes in muscle fibre diameter and in muscle fibre length. Oertel et al. (1988) studied postmortem sections of the human vastus lateralis and deltoid and noted an increase in mean fibre diameter from 10–12 μm shortly after birth to 40–60 μm between the ages of 15 and 20 years. Lexell et al. (1992) noted that the mean fibre diameter of the human vastus lateralis in postmortem specimens increases more than twofold between the ages of 5 and 20 years, and found that this was closely associated with a similar increase noted in muscle cross-sectional area. They noted an increase in the percentage of fast muscle fibres in the vastus lateralis from 35% at age 5 years to 50% at age 20 years: this was thought to represent a transition of muscle fibres from a slow to a fast phenotype. Increases in the amplitude of activation of muscle fibres may contribute to the increasing percentage of fast muscle phenotypes and the development of large MUs with growth, as although fast MHC isoforms are present at birth their subsequent expression depends on neurological innervation and hormonal factors (Agbulut et al., 2003). Longitudinal growth of the mouse gastrocnemius muscle belly occurs predominantly through muscle fibre hypertrophy rather than by increased muscle fibre length (White et al., 2010), but the situation in humans appears more complex. Binzoni et al. (2001) noted an increase in the pennation angle of the human medial gastrocnemius during growth which they felt reflected muscle fibre hypertrophy. Benard et al. (2011) used ultrasound to study the growth of the medial gastrocnemius in children aged between 5 and 12 years. They found that muscle fibre length and diameter increase with growth, but, because of the pennate nature of the gastrocnemius, longitudinal muscle fibre growth accounts for only 20% of the longitudinal growth of the medial gastrocnemius muscle belly: the remaining 80% was related to the increase in diameter of the muscle fibres. The contribution of muscle fibre growth in length and in diameter to longitudinal growth and increased volume of the muscle belly is likely to vary depending on the morphology of the muscle. Growth and development of muscle fibres is accompanied by development and organization of the connective tissue framework within the muscle, which results in an organized arrangement of muscle fibres within the muscle. Myostatin appears to be suppressed during perinatal and early postnatal muscle growth in rats, possibly to enhance development of the contractile component of muscle (Nishimura et al., 2007). Muscle fibre development and growth, the metabolic capacity of the muscle fibre and the development of the connective tissue network of muscle appear to reflect the interplay of a number of factors, including neuronal, nutritional and hormonal factors and the initial and subsequent pattern of muscle use. The mouse models of neonatal brachial plexus palsy noted above (Kim et al., 2009, 2010; Nikolau et al., 2011) suggest that the pattern of neuronal activation of muscle may be particularly important in postnatal muscle fibre growth and the development of the muscle phenotype. Muscle development and growth do not occur in isolation. The development of motor control appears to be facilitated by interaction of the musculoskeletal and corticospinal systems (Vinay et al., 2002). Forssberg (1985) reviewed human locomotor patterns in newborns (<2 months old), during supported locomotion (6–12 months old) and during early independent walking (10–18 months old) and noted co-contraction of agonists and antagonists. He suggested that innate pattern generators in the spinal cord produce the infant stepping, and also generate the basic locomotor rhythm in adults, but that neural circuits specific for humans transform the original, non-plantigrade motor activity to a plantigrade motor pattern. Sutherland et al. (1988) noted differences in the activation patterns of tibialis anterior (prolonged activity in stance), vastus medialis (prolonged activity in swing) and in the gastrocnemius and soleus (prolonged activity beginning near the middle of swing) in children 1–2 years old. These patterns gradually changed until by the age of 4 years a more mature pattern was seen in the majority of the muscles assessed. These changes appear to be associated with an improvement in the ability of the child to filter afferent input and to develop reciprocal inhibition. O’Sullivan et al. (1991) noted a gradual increase in the stimulus needed to invoke a monosynaptic reflex in typically developing children, increasing from a low level at birth to an adult level by the age of 6 years. The increase in threshold was associated with a reduction in activation of other muscles, including antagonists in the reflex response. Although we think of the development of motor control in terms of efferent output from the CNS, afferent input from muscles and afferent input generated by movement are also important: the development of a cortical motor map in the cat, for example, appears to depend on activity and motor experience (Martin et al., 2005). The refinement of the CST terminations and connections in the spinal cord, and of afferent fibres from muscle, appears to be driven by movement: blocking limb use in the cat between 3 and 7 weeks postnatally with the use of botulinum toxin resulted in a persistent abnormal morphology of CST axon terminals and a persistent prehension deficit (Martin et al., 2004). This may be related to competition between the CST, muscle afferent fibres and other inputs for cord synapses as suggested by Clowry et al. (2006) who noted similar findings in rats following the use of botulinum toxin in the second postnatal week to cause temporary limb paresis. These findings suggest close interaction between muscle development and function and continued motor development, with skeletal muscle acting as an important component of a feedback loop. Acquisition of the ability to stand, and subsequently take steps, requires appropriate motor control but also requires the presence of lower limb extensor muscles capable of producing sufficient force and with sufficient metabolic capacity to sustain generation of this force. An impairment of muscle development will result in an impaired capacity for movement and function, which may exacerbate any limitation in motor control. As will be discussed in the next section, there are a number of possible ways in which muscle development and growth may be altered in children with CP.
Early muscle development in children with cerebral palsy
the consequences for further muscle growth, muscle function, and long-term mobility
Skeletal muscle structure and function
Skeletal muscle changes in children with CP
Normal skeletal muscle development and growth
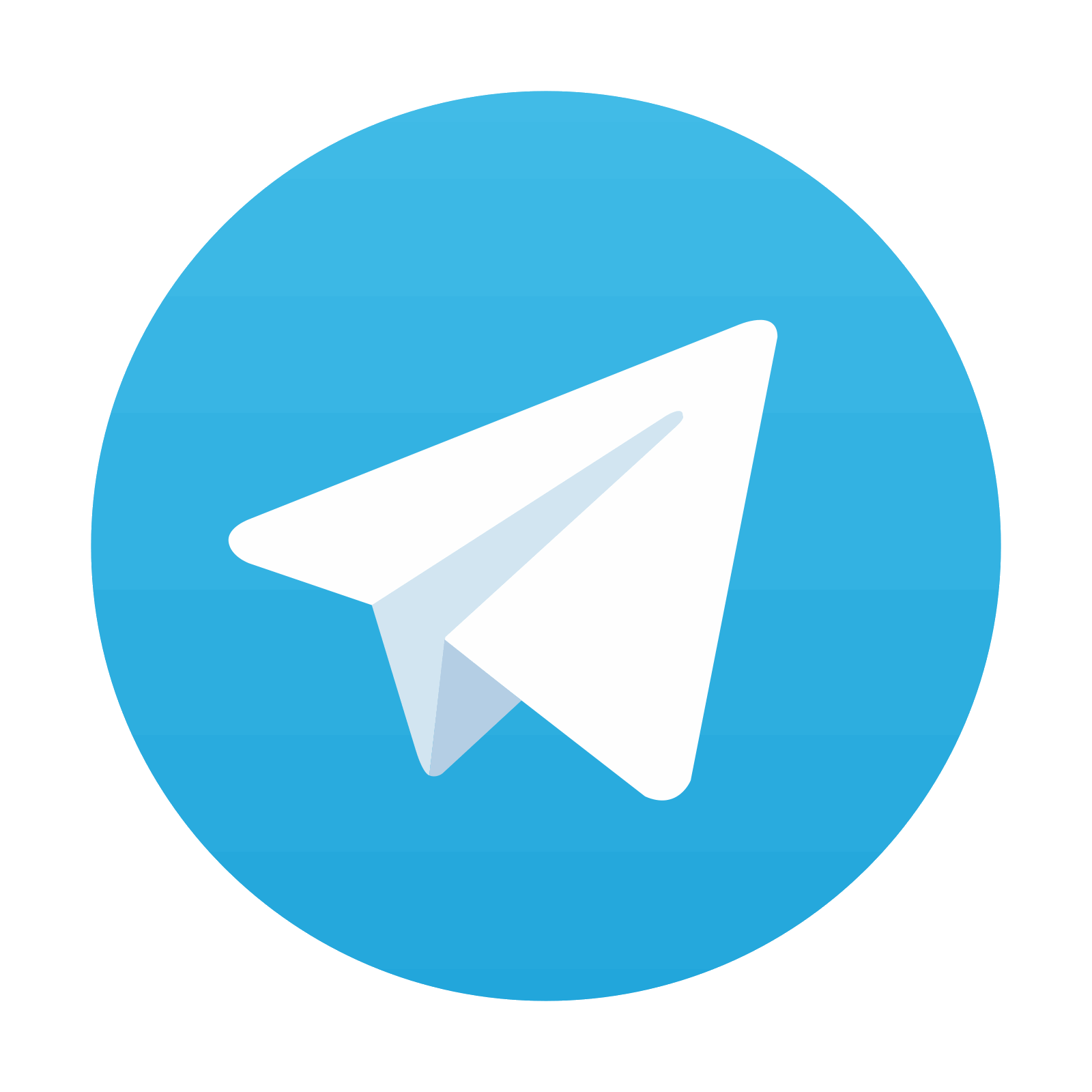
Stay updated, free articles. Join our Telegram channel
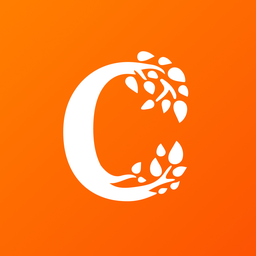
Full access? Get Clinical Tree
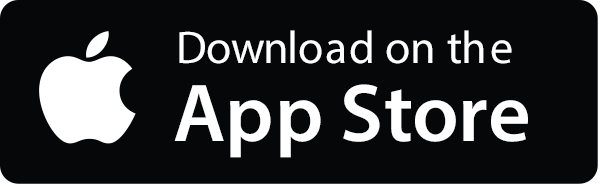
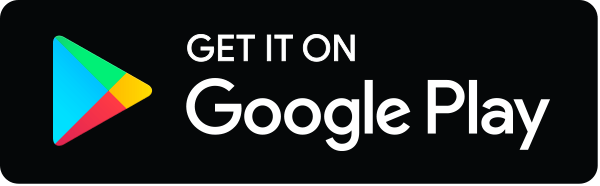