Fig. 3.1
Development of the notochord and nucleus pulposus. (a) The nucleus is derived from the notochord, a rod-shaped embryonic structure that lies under the neural tube. Sclerotome condenses around the notochord to form the vertebrae and annulus fibrosus of the intervertebral disc. Brachyury/T, Sd, Shh, and Sox5/6/9 are required for the formation of notochord and notochord sheath. (b) Once the vertebrae and disc start to form, the notochord contracts from the vertebral body and expands into the area of the future disc. The notochord sheath and collagen II, which helps to maintain osmotic pressure in the vertebral cartilage, are required for the expansion of the notochord and formation of the nucleus pulposus. (c) Growth and maintenance of the nucleus pulposus is in part controlled by the Skt gene
3.3.1 Development and Maintenance of the Notochord
Mutations in 19 genes resulting in absence of the notochord have been catalogued by the Mouse Genome Informatics (MGI) database (http://www.informatics.jax.org). The most well characterized is the T gene, encoding the brachyury protein (Kispert et al. 1994). Brachyury is the prototype T-box transcription factor. It is also considered a marker of primitive mesoderm and notochord. It starts out being expressed in all mesodermal cells. Later, it becomes restricted to the notochord where expression is maintained. Mice with homozygous mutations in T have defects in the formation of mesoderm, and the trunk notochord is not established (Dobrovolskaia-Zavadskaia 1927). Subsequent malformations in the spine and allantois lead to the early demise of the embryo. Mice with a dominant-negative mutation in T (Tc) survive but demonstrate abnormal nucleus pulposus morphology (Stott et al. 1993). Brachyury is also a marker for chordomas, rare malignant tumors along the spine thought to arise from persistent remnants of the notochord (Vujovic et al. 2006). Duplications in the T gene in humans have been associated with susceptibility to chordoma (Yang et al. 2009). For a complete discussion of chordomas, see Chap. 18.
While mice with mutations in T fail to establish the notochord, Danforth’s short-tail (Sd) mutation results in failure to maintain the notochord (Paavola et al. 1980). Sd mice arose from a spontaneous mutation in a yet unknown gene. Sd mice have abnormal nucleus pulposus morphology likely due to defects in the formation and maintenance of the notochord early in development. In Sd mice the notochord is discontinuous and becomes increasingly fragmented, eventually disappearing in mice homozygous for the mutant allele. In heterozygous mice, the intervertebral disc forms but the nucleus pulposus is absent and the disc is occupied with fibrous tissue similar to the annulus fibrosus (Semba et al. 2006).
Mutations in the Sickle tail gene (Skt), on the same chromosome as Sd, have nucleus pulposi; however, the nuclei are shifted to the periphery of the disc (Semba et al. 2006). The boundary between the nucleus pulposus and the annulus fibrosus is altered, and the annulus exhibits thin fibrous layers relative to control mice. The sequence of the Skt gene was identified using the gene-trapped ES clone from which it was derived. The protein product is predicted to have a proline-rich region in the N-terminus and a coiled-coil domain in the middle. The coiled-coil domain was similar to those found in a large number of scaffold proteins including keratins. It was suggested that while Sd is required at early stages of notochord development, Skt is required later in development for proper growth, differentiation, and maintenance of the nucleus pulposus. Furthermore, specific polymorphisms in the human SKT gene have been significantly associated with lumbar disc herniation in Japanese and Finnish case-controlled populations (Karasugi et al. 2009).
3.3.2 The Notochord Sheath and Formation of the Nucleus Pulposus
A sheath of extracellular matrix containing collagens and glycoproteins, including collagen II and laminin, surrounds the mesodermal cells of the notochord (Gotz et al. 1995). It has been proposed that the notochord sheath functions to contain and direct internal hydrostatic pressure within the notochord. The notochord is the forerunner of the axial skeleton in non-vertebrate chordates (Box 3.2). During early Xenopus development, osmotic inflation of the notochord against the sheath results in lengthening and straightening of the embryo (Adams et al. 1990). Similar mechanics-based mechanisms involving the notochord sheath may be involved in morphogenesis of the nucleus pulposus.
It has been shown that mutations in genes encoding proteins that affect the formation of the notochord sheath also affect the development of the nucleus pulposus (Smits and Lefebvre 2003; Choi and Harfe 2011). Sox5 and Sox6 are Sry-related HMG box transcription factors that cooperate with Sox9 to mediate chondrogenesis (Lefebvre 2002). Inactivation of Sox5 and Sox6 result in dramatic chondrodysplasia with impaired chondrocyte differentiation (Smits et al. 2001). Sox5/6 is also required for the formation of the notochord sheath and subsequent survival of the notochord and development of the nucleus pulposus (Smits and Lefebvre 2003). The sheath contains many matrix proteins that are also found in cartilage including collagen II and proteoglycans containing sulfated glycosaminoglycans like aggrecan and perlecan. The aggrecan and perlecan content in the notochord sheath from Sox5/6 mutant mice was dramatically reduced, whereas collagen II was not affected. It was suggested that Sox5/6 promotes the formation of the notochord sheath by regulating matrix gene expression in the notochord cells. Failure to maintain the sheath matrix resulted in premature death of notochord cells and an aberrant nucleus pulposus.
Shh, a secreted signaling molecule important for several aspects of development, is also required for the formation and maintenance of the notochordal sheath (Choi and Harfe 2011). Shh is secreted by the notochord as well as the nucleus pulposus in embryonic and postnatal life (Dahia et al. 2009; DiPaola et al. 2005). Shh binds to its receptor, Ptc, on nearby cells, thus relieving repression of Smoothened, Smo, a transmembrane protein that is required to transmit the Shh signal (Ingham and McMahon 2001). In mice containing a germ line null allele of Shh, the notochord was formed, but was not maintained (Chiang et al. 1996). The embryos died shortly thereafter preventing analysis of development of the intervertebral disc. To address the role of Shh in the maintenance of the notochord and the subsequent formation of the nucleus pulposus, a conditionally deleted allele of Smo in mice was used (Choi and Harfe 2011). In these experiments the investigators removed Smo from all Shh-expressing cells, including the notochord. The notochord sheath was missing in the Smo-deleted mice. Furthermore, the nucleus pulposus did not expand into the intervertebral disc region, and notochord cells were scattered throughout the vertebral column. Notochord remnants in the vertebral body could be marked by ROSA26 in ShhCreERT2 mice. If Smo was removed after the notochord sheath formed, the nucleus pulposus was not affected indicating the importance of the sheath in this tissue formation.
Collagen II (Col2) is the major collagen in cartilage and an important component of the notochordal sheath (Swiderski and Solursh 1992; Gotz et al. 1995). The notochord is not removed from vertebral bodies, and intervertebral discs do not form normally in mice with a null mutation in Col2a1 (Aszódi et al. 1998). Cartilage proteins including collagens IX and XI, aggrecan, and COMP appear to be expressed in these mice, but collagens I and II are inappropriately expressed in the cartilage of the presumptive vertebrae. As a result, the collagen fibers in cartilage are disorganized. It was proposed that this disorganization would lead to a weakened structure unable to contain the cartilage osmotic pressure. The reduction in internal pressure within the vertebral body could be responsible for the failure of the notochord to be removed from the vertebrae and expand into the intervertebral disc (Adams et al. 1990), as a result, the nucleus pulposus would not form. Mutations in other genes that are expressed in the sclerotome and affect the development of the vertebral body including Nkx3.2 and Pax1/9 also affect the removal of the notochord from the vertebral body and development of the nucleus pulposus, further supporting the requirement of mechanical pressure for removal/expansion of the nucleus pulposus (Peters et al. 1999; Lettice et al. 1999; Tribioli and Lufkin 1999).
3.3.3 Box 3.2: Evolution of the Intervertebral Disc
The notochord is the forerunner of the axial skeleton in early non-vertebrate chordates. In basal vertebrates including lamprey and fish (teleosts), most of the axial skeleton is derived from the notochord (Koob and Long 2000; Ytteborg et al. 2012; Haga et al. 2009; Dale and Topczewski 2011; Inohaya et al. 2007). Sclerotome is not detected in non-vertebrate chordates. A primitive sclerotome, however, is observed in the most basal vertebrates and teleosts (Scaal and Wiegreffe 2006; Keller 2000; Koob and Long 2000; Inohaya et al. 2007). Development of the axial skeleton in teleosts, including salmon, medaka, and zebra fish, begins with patterned mineralization of the notochord sheath where the vertebrae will form (Ytteborg et al. 2012; Haga et al. 2009; Dale and Topczewski 2011; Inohaya et al. 2007). In adult fish, the disc consists of a central core, similar to the nucleus pulposus, containing cells with large fluid-filled vacuoles derived from the notochord, a fibrous layer derived from the notochord sheath, and an elastic membrane or ligament that is likely derived from the primitive sclerotome (Ytteborg et al. 2012; Haga et al. 2009; Dale and Topczewski 2011; Inohaya et al. 2007).
Comparative anatomy suggests the sclerotome evolved further in amphibians. In some, the sclerotome composes a large portion of the differentiating somite, and segmentation is independent of the notochord (Scaal and Wiegreffe 2006; Keller 2000). By the time amniotes evolved, they already had a well-developed intervertebral disc with a nucleus-like tissue derived from the notochord and an annulus-like fibrous tissue derived from an evolving sclerotome. It was recently shown that some adult reptiles and birds, including chicken and quail, lack a nucleus pulposus and there is limited, if any, contribution of the notochord to the adult axial skeleton (Bruggeman et al. 2012). Since the contribution of the notochord to the axial skeleton and development of a nucleus pulposus-like structure occurred very early in vertebrate evolution, before significant contribution of sclerotome derived tissues, it seems likely that the nucleus pulposus was lost during the evolution of reptiles and birds. This loss could have been due to either selective pressure to lose the nucleus pulposus or lack of pressure to keep it. Perhaps as the sclerotome evolved, it was able to take over all of the functions of the adult axial skeleton that were previously served by the notochord.
The line that would lead to mammals (synapsids) and that of reptiles and birds (saurapsids) diverged from the basal amniotes some 320 million years ago. Whatever the reason (pressures related to gait or mechanical loading), mammals retained the notochord-derived structure. Of course, proof of this model will require additional fossil evidence, which is scarce for unmineralized tissue; more complete comparative anatomy of existing species; and further experimentation, including lineage tracing and molecular biology, on the development of the intervertebral disc in multiple species.
3.3.4 Evidence for Lineage of the Nucleus Pulposus
The lineage of the cells in the adult nucleus pulposus has been the source of much debate (Risbud et al. 2010; Erwin 2010; Shapiro and Risbud 2010). It has been proposed that the notochord forms the initial central part of the intervertebral disc, but as this compartment matures, these large notochord cells are replaced with smaller cells that more closely resemble chondrocytes, perhaps recruited from the end plate or the inner annulus (Wamsley 1953; Kim et al. 2003). However, more recently, evidence has accumulated to suggest that all of the cells within the nucleus pulposus are indeed derived from the notochord.
Shh is highly expressed in the notochord and later in the nucleus pulposus (Dahia et al. 2009; DiPaola et al. 2005; Choi et al. 2008). Mice that express an inducible Cre under the control of the Shh promoter were used in fate mapping studies of the notochord (Choi et al. 2008). To exclude the possibility that Shh-Cre would mark cells in the notochord and then again in a new nucleus pulposus cell population, a tamoxifen-inducible Cre was employed. Notochord cells were “pulse labeled” by administration of tamoxifen at an early stage of development and then followed over time with ß-galactosidase staining through Cre-mediated activation of the ROSA26-LacZ locus. When mouse embryos were pulse labeled at E8.0 days of gestation and examined at E13.5, it was clear that all of the cells of the nucleus pulposus were labeled. Furthermore, when old mice (19 months) were examined, all of the cells of the nucleus pulposus were labeled with no labeling observed in the annulus fibrosus. More recently, notochord cells were traced using a Noto-Cre mouse (McCann et al. 2012). Noto is a highly conserved transcription factor with expression limited to the node and early notochord. Again, when cells were marked by activation of the ROSA26-LacZ locus by Noto-Cre, all of the nucleus pulposus cells in the adult, after dramatic changes in cellular morphology, were still labeled although only a subset of adult nucleus cells stained for K8, a putative marker for nucleus pulposus cells. The fate mapping studies strongly suggest the nucleus pulposus is derived completely from the notochord.
Evidence that the nucleus pulposus is derived from the notochord also comes from gene profiling studies. Recent studies used microarray technology to compare the gene expression patterns in human, bovine, canine, and rodent nucleus pulposus, annulus fibrosus, and articular cartilage (Minogue et al. 2010; Lee et al. 2007; Sakai et al. 2009). Brachyury/T, K8, K18, and K19 were highly expressed in the nucleus pulposus. Brachyury/T is known as a marker of the notochord and also a marker of chordoma, notochord tumors, suggesting that the nucleus pulposus could be derived from these embryonic cells. More importantly, gene expression patterns significantly overlapped, and expression of the above nucleus pulposus markers was similar in the large notochord-like cells and the smaller chondrocytic cells within the nucleus pulposus. In contrast to this result, another group showed heterogeneity in the nucleus pulposus with regard to K8 expression (Gilson et al. 2010). Heterogeneity in K8 expression was also observed in the Noto-Cre cell fate mapping studies described above, even though all of the nucleus pulposus cells were clearly derived from Noto-expressing cells (McCann et al. 2012). A likely explanation is that the notochord can differentiate into all of the cell types including K8-expressing and K8-non-expressing cells, within the nucleus pulposus. This idea is supported by studies showing that notochord cells from rabbit can differentiate into cells with varying morphological characteristics similar to those observed in the adult nucleus pulposus (Kim et al. 2009). Taken together, there is now considerable evidence indicating that all of the cell types in the adult nucleus pulposus are derived from the notochord.
3.4 Somitogenesis
Somites are transient structures formed from the presomitic mesoderm (PSM) that define the anterior-posterior segmented pattern of the embryo. They are essentially balls of cells composed of an outer epithelial layer surrounding a mesenchymal core, the somitocoele (Ferrer-Vaquer et al. 2010). The formation of somites is tightly controlled during development both spatially and temporally. Somite pairs bud off from the anterior end of the PSM at time intervals specific to the species (Pourquie 2011; Brand-Saberi et al. 2008). Somite nomenclature is based on the relationship of the somite to the anterior end of the PSM with the closest somite labeled number SI followed by SII, SIII, etc., counting toward the anterior end of the embryo (Christ and Ordahl 1995). Future somites in the presomitic mesoderm are labeled S0 and SI. These somites have not budded off the PSM, but can be seen histologically or with molecular markers and are frequently referred to as somitomeres. It is important to note that the first five somites to bud off are destined to fuse and form the occipital bone (Couly et al. 1993). The remaining somites will progress into the components of the axial skeleton and skeletal muscle. Disruptions to somitogenesis and segmentation of the somites result in birth defects that can severely affect the function of the spine by disrupting the formation and shape of both vertebral bodies and intervertebral disc (Box 3.3; Turnpenny 2008).
3.4.1 Box 3.3: Human Pathologies Associated with Segmentation Defects: Klippel-Feil Syndrome
Many congenital defects of the spine are caused by problems in segmentation of the somites (Turnpenny 2008; Shifley and Cole 2007). Some of these are linked to teratogens such as retinoic acid (RA) which can cause axial skeleton abnormalities by interfering with the normal retinoid signaling and thus affecting the normal timing and location of segment formation (Alexander and Tuan 2010). Various types of spondylocostal dysostosis have been linked to the clock genes Dll3, Lnfg, Hes7, and Mesp2 (Pourquie 2011). Alagille syndrome, which is characterized by misshapen “butterfly” vertebrae caused by dorsal fusion failure, has been linked to mutations in Jagged1, a Notch ligand (Oda et al. 1997; Li et al. 1997). Segmentation defects can also affect the intervertebral disc as well as the vertebra. Most prominently, the disc is completely absent with fused block vertebrae and pushed to one side when only partial vertebral fusion occurs (Turnpenny 2008; Shifley and Cole 2007). Unfortunately, many clinical observations fail to include discal analysis, most likely due to inability to visualize it by earlier imaging methods.
Klippel-Feil syndrome (KFS) is one of the clearest examples of a disc development defect in humans. KFS is primarily characterized by congenital cervical synostosis due to an absence of the disc and resulting in fusion of adjacent vertebrae. First identified in 1912 by Klippel and Feil, it was described as limited head range of motion, low posterior hairline, and absence of neck (Klippel and Feil 1975; Willard and Nicholson 1934). KFS is currently categorized into three types as follows (Tracy et al. 2004; Kaplan et al. 2005):
Type I – multiple cervical fusions
Type II – 1–2 cervical fusions
Type III – cervical fusion combined with lumbar fusions
After a screen of 63 affected individuals showed 6 with potential deleterious mutations in PAX1, it was proposed that defects in PAX1 function resulting in somite segmentation defects were the major cause of KFS (McGaughran et al. 2003). More recently, genetic analysis of both inherited and sporadic cases of KFS identified two missense mutations (L298P and A249E) in the GDF6 gene and an inversion (q22.2q23.3) 623 kb 3′ of GDF6 (Clarke et al. 1995; Tassabehji et al. 2008). GDF6 (also known as BMP13) is a member of the TGF-ß protein family that clusters with GDF5 and GDF7 (BMP14, BMP12) and is structurally related to BMP2 and BMP4 (Rider and Mulloy 2010). Knockdown of GDF6 in zebra fish and Xenopus results in KFS-like phenotypes (Asai-Coakwell et al. 2009; Tassabehji et al. 2008). Deletion of GDF6 in mice does not result in any obvious spinal phenotypes; however, the additional deletion of GDF5 results in a KFS-like scoliosis phenotype suggesting some redundancy in the function of GDF5 and GDF6 (Asai-Coakwell et al. 2009; Settle et al. 2003). It is also important to note that even with the double deletion, these mice do not exhibit vertebral fusions. It has been shown that GDF6 can inhibit endochondral ossification of mesenchymal stem cells in culture suggesting that GDF6 may normally help to specify or maintain the disc space in the sclerotome (Shen et al. 2009). Furthermore, it has been shown that GDF6 prevents the effects of annular injury in an ovine model and may act as a protective agent for the annulus fibrosus (Wei et al. 2009). These only cover a few of the many abnormalities associated with segmentation, but future genetic screening promises to link more cases with the genes involved in somitogenesis.
3.4.2 Clock and Wavefront
The first step in the formation of the somite from the PSM is the determination and timing of the segmentation site. This anterior-posterior segmentation and patterning of the PSM was originally hypothesized by Cooke and Zeeman (1976) to occur via a “clock and wavefront” mechanism (Fig. 3.2). This model proposed that the tightly controlled size and number of somites is due to the cycling of expression for specific genes within the PSM cells. Cycling of gene expression within individual cells provides the “clock,” and the presence of a morphogen gradient across the anterior-posterior axis of the embryo provides the “wavefront.” This gradient in combination with the timing of genes expressed through the clock leads to the formation of a boundary where the somite will separate from the PSM. Commitment and differentiation of cells is also modulated as they pass through this boundary. Additional experimental evidence over the years has supported and expanded upon the “clock and wavefront” model so that now many of the molecular details have been elucidated (Pourquie 2011; Brand-Saberi et al. 2008).
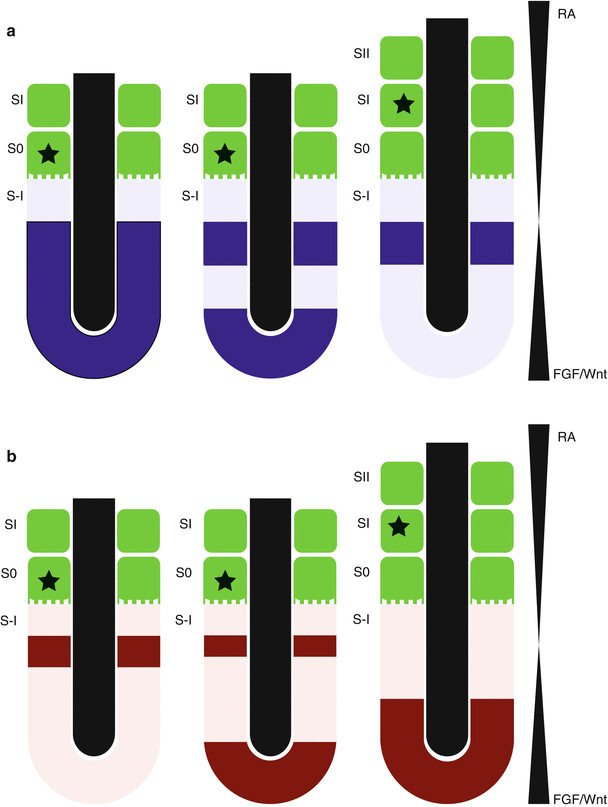
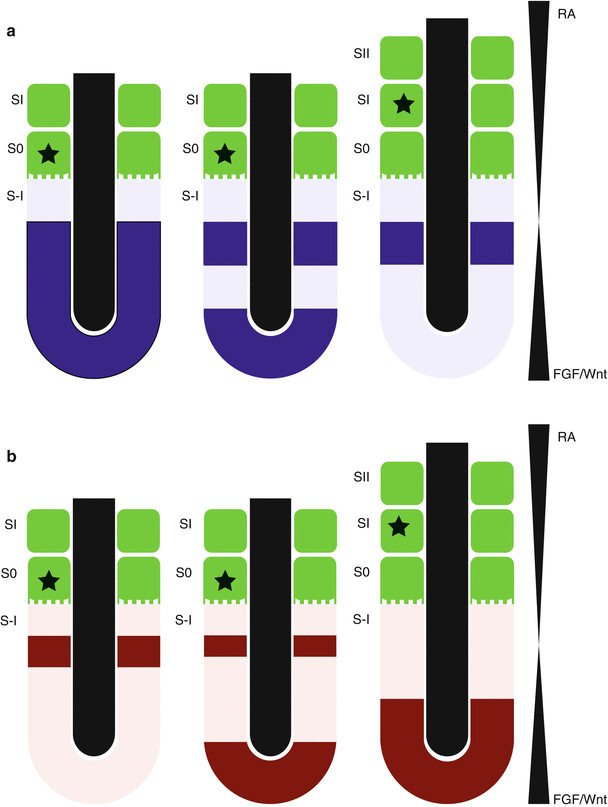
Fig. 3.2
Somitogenesis (segmentation). Somites (green) bud off at evenly spaced time intervals, the period of which is determined by species, from the anterior PSM. Counter gradients of RA and FGF/Wnt signaling determine the location of the determination front near the posterior boundary of somite SI. The two main “clock” signaling pathways cycle on and off synchronously with somite formation yet slightly out of phase with each other. (a) Blue stripes are representative of Wnt pathway gene cycling within the PSM during the period of one somite generation. (b) Red stripes are representative of Notch pathway gene cycling within the PSM during the period of one somite generation. The star indicates the position of a single cell during the period of formation for one somite
3.4.2.1 Clock Regulation
Two major signaling pathways are involved in regulating the “clock” that helps determine where and when each somite will separate from the PSM. The first is the Notch pathway. Notch1 has a critical role in the segmentation and epithelialization of somites (Conlon et al. 1995; Swiatek et al. 1994). Although Notch1 is expressed throughout the PSM, activated Notch signals where segmentation will occur (Reaume et al. 1992) and other pathway members that regulate Notch activity, such as delta1(Dll1) (Bettenhausen et al. 1995), Hes1 (Palmeirim et al. 1997; Dequeant et al. 2006), Hes7 (Bessho et al. 2003), and lunatic fringe (Lfng) (Evrard et al. 1998; Johnston et al. 1997; Forsberg et al. 1998; Zhang and Gridley 1998), exhibit cyclic expression patterns in the PSM. The pattern of expression has been compared to waves washing up on shore. Expression starts at the posterior end of the PSM and moves in a wave to the point where the next somite will form, then expression starts back at the posterior domain. The timing of the cycle is species dependent. Mesp2, an inhibitor of Notch signaling, also has a cyclic expression pattern in the PSM, and its expression becomes restricted to, and maintained in, the anterior portion of the SI somite at each cycle (Morimoto et al. 2005). This sets up a sharp boundary of Notch activity that is high in the posterior end of the S0 somite and low in the anterior end of the future SI somite, thus defining the segmentation site for the S0 somite (Morimoto et al. 2005; Saga 2007; Sasaki et al. 2011; Saga et al. 1997).
The second signaling pathway shown to be involved in the clock system and in regulating its cyclic pattern of gene expression is that of Wnt. Axin2, a negative regulator of the Wnt pathway, has an oscillating pattern of expression that is not in synchrony with genes of the Notch family (Aulehla et al. 2003; Dequeant et al. 2006). Disruption of the Notch pathway does not disrupt Axin2 expression, although disruption of Wnt3a disrupts Lnfg, an inhibitor of Notch signaling (Aulehla et al. 2003, 2008). This suggests that cyclic Wnt signaling is occurring upstream of the cyclic Notch signaling. This cycling of gene expression seems to allow the cells of the PSM to respond to the “wavefront” signals at the appropriate time initiating the cellular shape and adhesion changes that permit the somite to “bud off” from the PSM.
3.4.2.2 Wavefront
The concept of the “wavefront,” a point of abrupt transition leading to somite formation, has evolved to include two counteracting morphogen gradients (Brand-Saberi et al. 2008; Pourquie 2011). The first identified was that of fibroblast growth factor (FGF) signaling. FGF8 was found to be present at high levels in the posterior PSM and gradually decreases toward the anterior PSM (Dubrulle et al. 2001). More recently, FGF4 was seen expressed in a similar pattern (Naiche et al. 2011). Experiments implanting beads soaked in Fgf8 have demonstrated the ability of Fgf8 concentration to regulate the size of the developing somites (Sawada et al. 2001). Deletion of Fgfr1, the only FGF receptor expressed in the PSM, results in loss of cyclic gene expression and failure of somite segmentation (Wahl et al. 2007). The FGF8 gradient has been determined to be due to the instability of the RNA transcripts that leads to a gradual decrease in Fgf8 protein (Dubrulle and Pourquie 2004), thus allowing for the tight temporal and spatial control of the segmentation boundary. In addition to FGF, Wnt signaling shows a similar gradient, as evidenced by localization of β-catenin protein, that acts both through and parallel to FGF signaling (Aulehla et al. 2008). This Wnt signaling gradient also suggests a mechanism of cross talk between the “clock” and “wavefront” as Wnt signaling can control the Notch pathway. It has been suggested that the gradient and cyclic expression of Wnt acts as a bridge between the wavefront and clock (Brand-Saberi et al. 2008; Pourquie 2011).
The counter gradient to FGF is a retinoic acid (RA) gradient that extends in an anterior to posterior direction along the developing embryo and is inhibited by Fgf8 through repression of Raldh2, a gene required for RA synthesis (Diez del Corral et al. 2003). In turn, RA is then able to repress FGF signaling (Diez del Corral et al. 2003). Cyp26, an enzyme that degrades RA, is expressed in the tail bud (Sakai et al. 2001) providing a mechanism to keep the diffusion of RA localized. This method of gradient formation is known as a source-sink mechanism (Aulehla and Pourquié 2010) in contrast to the mRNA decay used to control the FGF gradient. It is currently thought that the boundary of the “wavefront” is determined by the transition from high FGF/Wnt and low RA signaling to low FGF/Wnt and high RA signaling.
The bilateral symmetry of the somite pairs produced during somitogenesis is also controlled by RA signaling (Kawakami et al. 2005; Vermot et al. 2005; Vilhais-Neto et al. 2010; Vermot and Pourquie 2005; Sirbu and Duester 2006). During normal embryonic development, the asymmetric expression of various genes, such as Nodal and Pitx2, occurs directing the organization of the internal organs. It has been proposed that RA acts to insulate somitogenesis from surrounding signals driving the left-right asymmetry seen throughout the body cavity (Brent 2005; Brend and Holley 2009). This work links the genes involved in somitogenesis directly to the control of body symmetry.
3.4.3 Epithelialization
As somites bud off of the anterior end of the PSM, the outer cell layer undergoes a mesenchymal to epithelial transition (MET). The two primary transcription factors involved in MET are Pax3 and Paraxis. In vitro overexpression of Pax3 results in epithelialization of mesenchymal cell lines (Wiggan et al. 2002), while deletion of Pax3 in the PSM results in somites that are unable to maintain epithelial integrity (Mansouri et al. 2001). Loss of Paraxis also disrupts epithelialization of the somite (Burgess et al. 1996) although it does not affect segmentation and future differentiation. The first evidence for MET during somitogenesis came from a finding in 1978 that cells from the segmented mesoderm showed a greater adhesiveness than those of the unsegmented PSM (Bellairs et al. 1978). Various adhesion molecules are expressed in the developing somite such as N-cadherin, fibronectin, cytoactin, and neural cell adhesion molecule (Duband et al. 1987; Crossin et al. 1986). These molecules are known to be involved in the formation of epithelia. N-cadherin is necessary for the production of stable somites with its loss resulting in irregular and loosely attached somites (Radice et al. 1997) as well as lack of adhesion in culture (Duband et al. 1987). EphA4/Ephrin signaling is also required for proper epithelialization of the somite. Ablation of EphA4/Ephrin signaling in the form of a dominant-negative, truncated EphA4 results in somite boundaries, but no epithelial layer formation (Barrios et al. 2003). This process results in epithelia forming only on the outer boundary of each somite sphere. It is not clear how high Notch signaling from the segmentation clock translates into MET, but Notch regulates the expression of a transcription factor called Hes1 that regulates Ephrin expression (Glazier et al. 2008).
3.4.4 Segment Identity
Hox genes control the patterning of the PSM, which will ultimately lead to the differences in cranial to sacral vertebrae identity (Iimura et al. 2009; Wellik 2009). Originally discovered in drosophila segmentation, Hox genes are located in clusters that are arranged from 3′ to 5′ in the order of their expression from anterior to posterior domains. In mammals, there are four clusters Hox A, B, C, and D each containing up to 13 Hox genes (Wellik 2009). Hox genes with the same number across clusters are called paralogous groups (e.g., Hoxa1, Hoxb1, and Hoxd1). The expression domains of individual Hox genes have been shown to line up with specific vertebral segments (Burke et al. 1995). Although the colinearity of the Hox genes is easily demonstrated in drosophila with deletions resulting in anterior homeotic transformations, in the mouse, Hox deletions result in both the expected anterior homeotic transformations and posterior homeotic transformations (Wellik 2007). The reason for this variation seen in mice is thought to be due to the activity of paralogous Hox genes. Deletion of multiple paralogous Hox genes consistently results in anterior homeotic transformations (Wellik 2007). The term “Hox code” is used to describe the phenomenon in which a very specific complement of Hox expression determines the identity of a specific vertebral segment (Kessel and Gruss 1991; Iimura et al. 2009). In the mouse, the anterior boundaries of Hox gene expression are set by embryonic day 12.5 (Wellik 2007). There has been some indication that Hox gene expression is linked to the somite clock (Zakany et al. 2001; Kmita and Duboule 2003) allowing additional layers of specification in the patterning of the spine. RA has also been linked to the control of Hox gene expression during specific stages of development with ectopic RA causing homeotic transformations in the vertebrae (Kessel and Gruss 1991). Thus, there is cross talk between the signaling pathways in the “wavefront” and those required for location specification. This activity emphasizes how all of the signaling pathways that regulate development of the axial skeleton overlap and intertwine.
3.5 Formation of Sclerotome
Differentiation begins starting with the anterior-most somite. The dorsal epithelium of the somite will form the dermomyotome, which will later differentiate into the dermis of the back and skeletal muscle. The somitocoele and the ventral epithelium of the somite will undergo an epithelial to mesenchymal transition (EMT) to generate sclerotome, which will form all of the connective tissues of the axial skeleton. The division of sclerotome and dermomyotome begins when the somite reaches SIII (Christ and Ordahl 1995) although the sclerotome segment is still plastic until a much later stage (Dockter and Ordahl 2000). Shh secreted from the notochord and ventral floor plate is the primary inductive signal controlling the delamination of the epithelial somite to form the sclerotome (Borycki et al. 1998; Fan and Tessier-Lavigne 1994; Murtaugh et al. 1999; Dockter 2000; Chiang et al. 1996; Marcelle et al. 1999). Pax3 and Pax7 are expressed in the unsegmented PSM but are downregulated in the ventral somite and somitocoele as the sclerotome differentiates. The expression of Pax1 and Pax9, markers of sclerotome, increases (Brand-Saberi and Christ 2000). Shh has been shown to induce Pax1, a marker of sclerotome, when present ectopically (Fan and Tessier-Lavigne 1994; Johnson et al. 1994). A second permissive signal is also required for the formation of sclerotome (Stafford et al. 2011). BMP from the lateral plate mesoderm normally interrupts differentiation of sclerotome and interferes with Shh signaling, allowing the sclerotome to differentiate only from the ventral medial side of the somite. Several extracellular BMP antagonists are expressed regionally that carefully restrict BMP activity (Stafford et al. 2011; Rider and Mulloy 2010). Noggin (Nog) and Gremlin1 (Grem1) cooperate to antagonize BMP signaling, permitting sclerotome differentiation in the presence of Shh (Stafford et al. 2011). Deletion of Nog and Grem1 in mice results in the complete failure of sclerotome differentiation. Dermomyotome was not affected in these mice. Inhibition of BMP alone is not sufficient to specify sclerotome or expand sclerotome differentiation in vivo suggesting antagonism of BMP is a permissive factor for sclerotome differentiation (Rider and Mulloy 2010). Very recently, using notochord deficient Sd mice, it was shown that the floor plate alone is sufficient for the development of the sclerotome (Ando et al. 2011). Shh from the floor plate could replace Shh from the notochord to allow differentiation of sclerotome in the absence of notochord.
3.6 Resegmentation
Resegmentation of the sclerotome was first proposed by Remak in 1855 (Bagnall et al. 1988) after observing that in relationship to the original somites, there was half-segment realignment of the vertebrae. The initial studies followed somite development in chick embryos through lineage tracing using quail/chick chimeras (Bagnall et al. 1988; Goldstein and Kalcheim 1992), vital dye (Bagnall 1992), or viral transduction (Ewan and Everett 1992). The consensus from these studies was that the sclerotome derived from one somite divides into rostral and caudal halves with the rostral half of one segment joining with the caudal half of the segment immediately rostral to it forming the vertebral body. Accordingly, the intervertebral disc would be derived from the sclerotome at the junction of the two half segments (Fig. 3.3). Since those early reports, it has been determined that resegmentation occurs after the sclerotome differentiates from the ventral portion of the somite. Resegmentation results in a one half-segment stagger between the sclerotome and myotome allowing for the alternating pattern between the musculature and the vertebral body in the axial skeleton. Phenotypes indicative of alterations in resegmentation include fusion of vertebrae where joints should be, split vertebrae and ribs, alterations in migration of neural crest through the sclerotome and disorganization of the dorsal root ganglia. Several mouse models demonstrate these types of resegmentation defects including deficiencies in RAB23 (opb mutant) (Sporle and Schughart 1998), Paraxis (Johnson et al. 2001), and Tgfbr2 (Baffi et al. 2006). Ablation of the neural tube after sclerotome formation also results in resegmentation failure suggesting a role for signals from the neural tube in directing resegmentation (Colbjorn Larsen et al. 2006). Much of the molecular mechanisms defining the process of resegmentation remain to be elucidated.
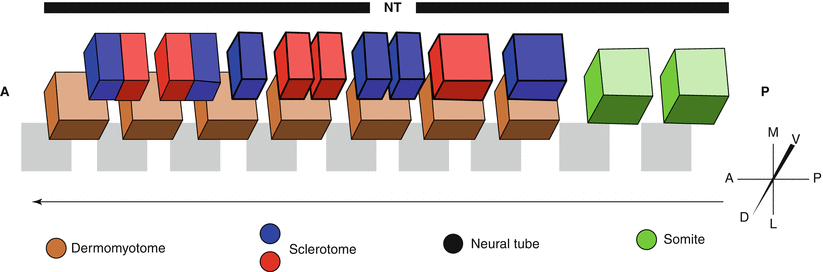
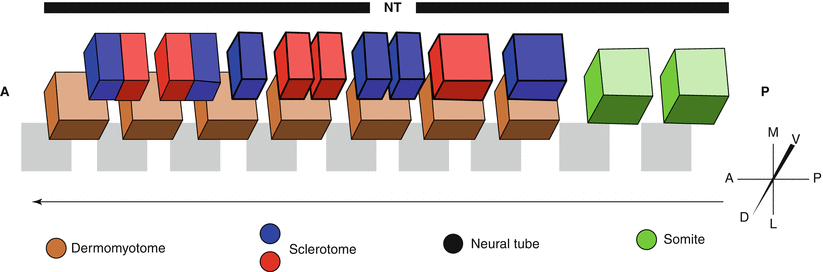
Fig. 3.3
Sclerotome resegmentation. Resegmentation begins with the dorsal-ventral division of the somite into the dermomyotome (brown) and sclerotome (red and blue), respectively. The sclerotome then proceeds to divide into anterior and posterior regions that can be seen as alternating loose and condensed mesenchyme. The anterior region of one sclerotome then associates with the posterior region of the sclerotome directly anterior to form what will later become an individual vertebral body. In this way the vertebral body and muscle segment will be staggered by one half segment. Arrow indicates direction of cell fate determination. NT Neural Tube
3.7 Sclerotome Derivatives
Sclerotome contains multipotent progenitor cells that differentiate into all of the connective tissue cell types of the axial skeleton (Monsoro-Burq 2005). Sclerotome can differentiate into cartilage that will subsequently undergo endochondral ossification to form the bony vertebrae of the spine. It will also differentiate into the annulus fibrosus of the intervertebral disc and the tendons that link the vertebrae to the muscle. Which cell type is specified is determined by the location of cells within the sclerotome and complex interactions of growth factors (Fig. 3.4). How these factors interact with each other to specify cell lineage in the axial skeleton is just beginning to be determined.
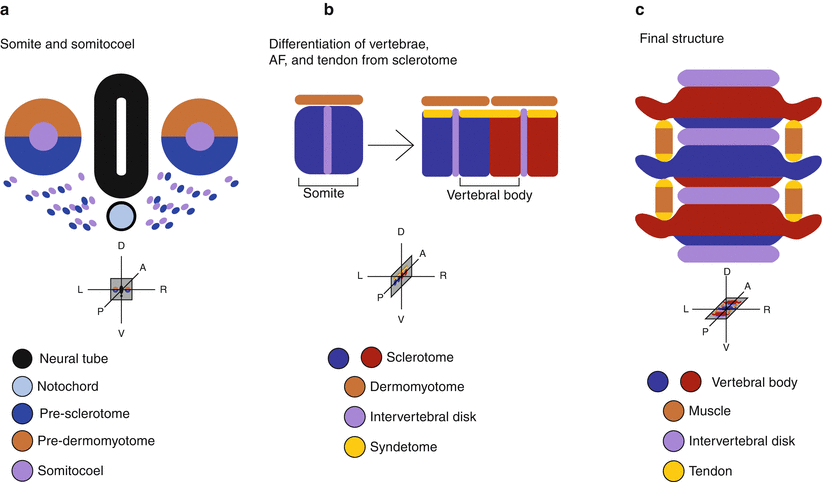
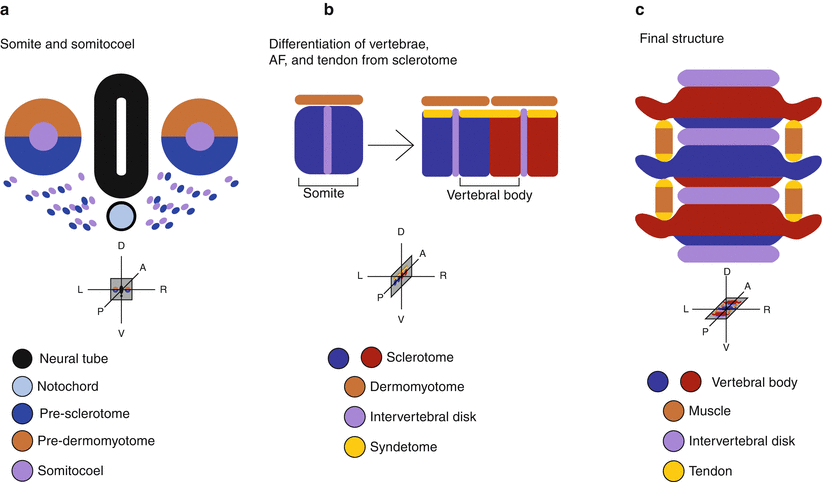
Fig. 3.4
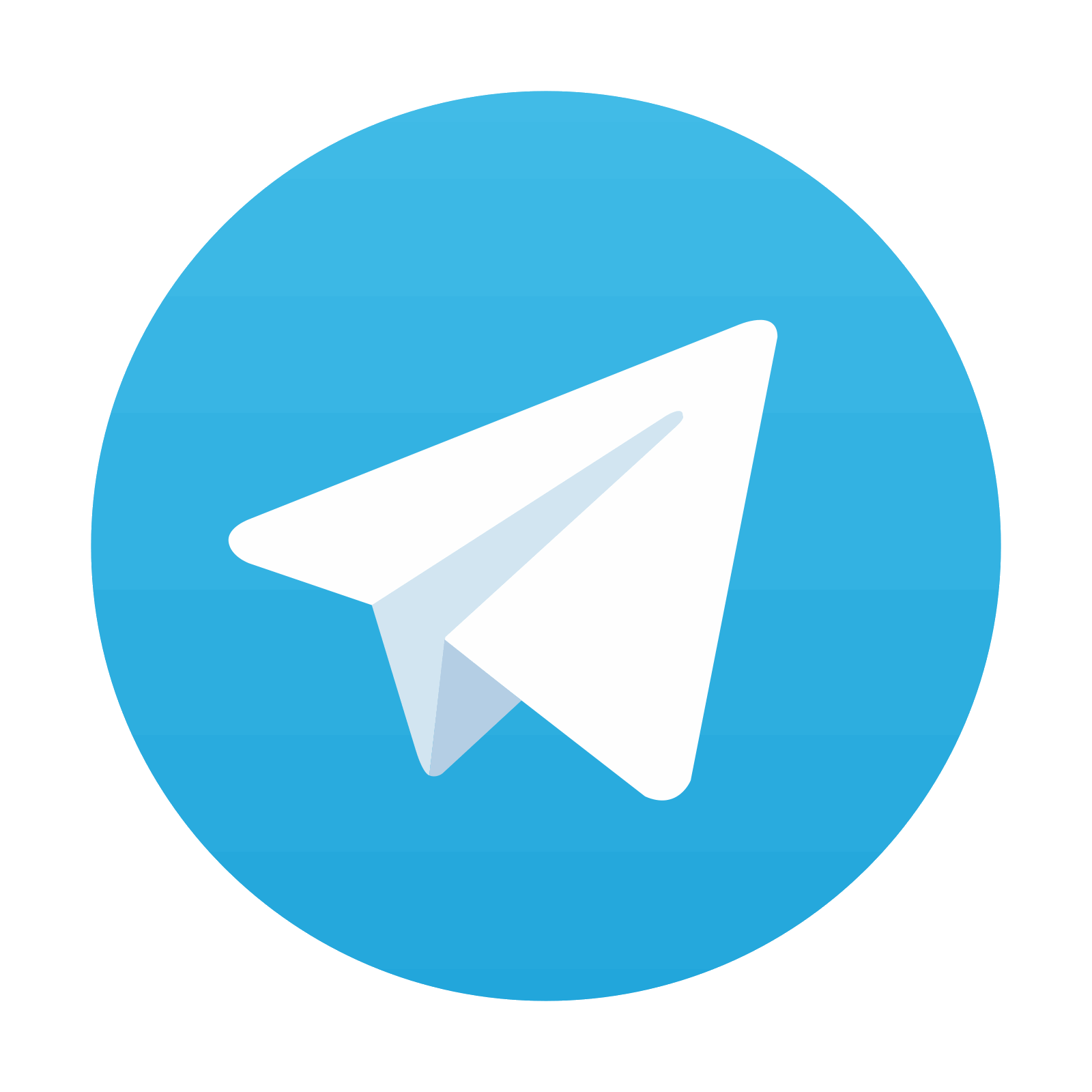
Derivatives of the sclerotome. (a) Sclerotome is derived from the ventral medial portion of the somite. In response to Shh, cells from the somite and somitocoele migrate toward the notochord and differentiate into sclerotome. (b) Cells from the somitocoele end up at the border between the rostral and caudal halves of each segment. After resegmentation, these cells lie in between the developing vertebral bodies and will become the annulus fibrosus (AF) of the intervertebral disc. Cells at the dorsal border of the sclerotome differentiate into tendon in response to factors from the myotome. (c) In the final structure, the disc is situated between the vertebral bodies, which are staggered by one half segment from the muscle and connected to the muscle though the tendons
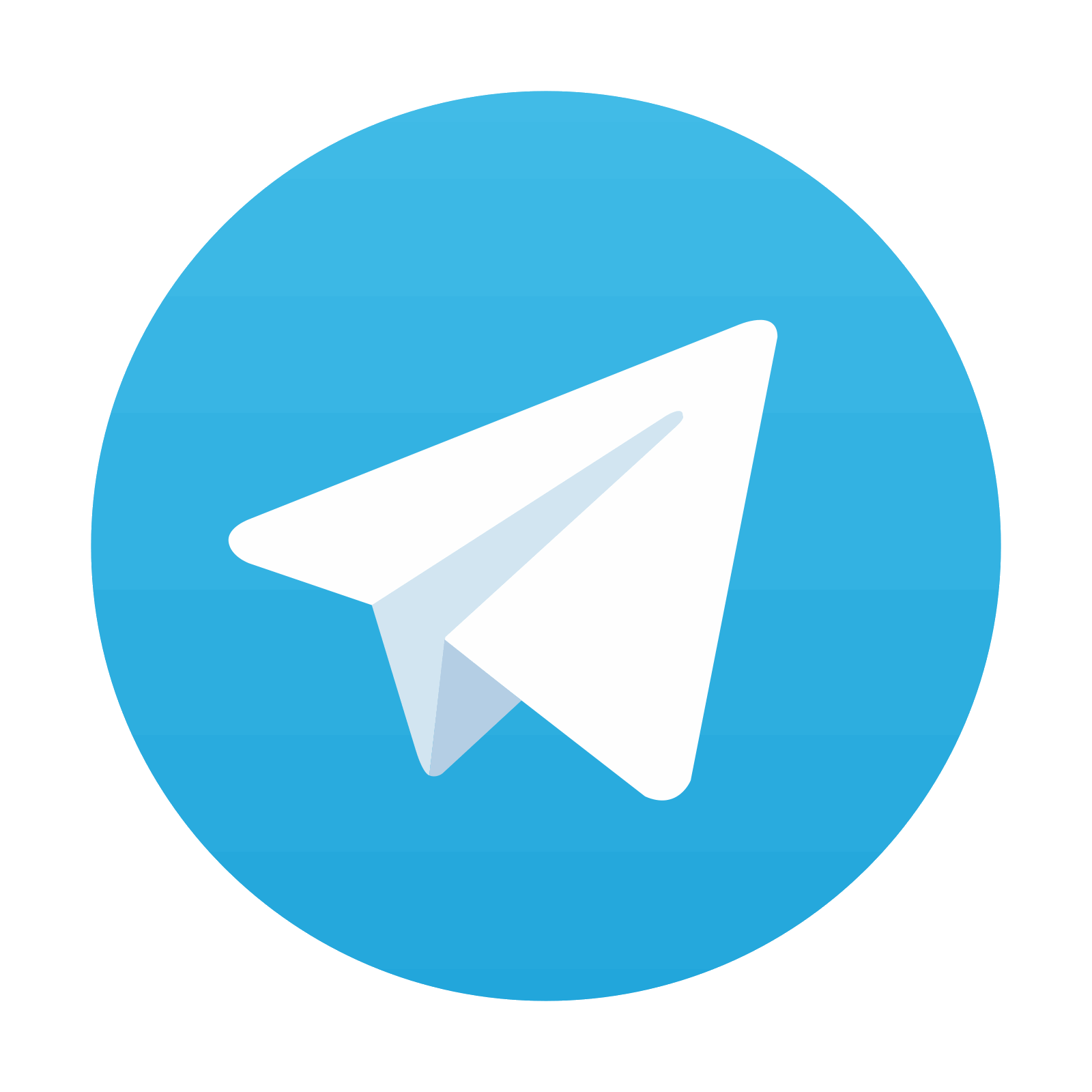
Stay updated, free articles. Join our Telegram channel
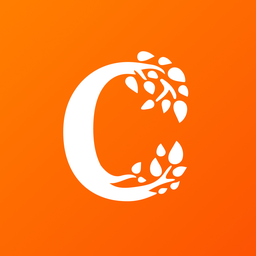
Full access? Get Clinical Tree
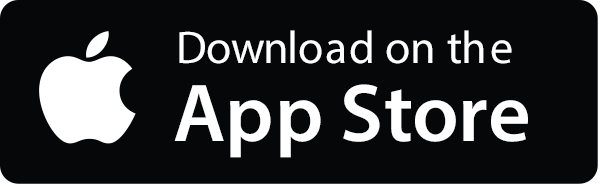
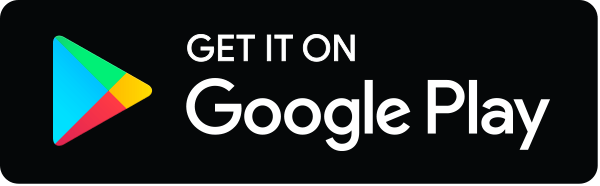
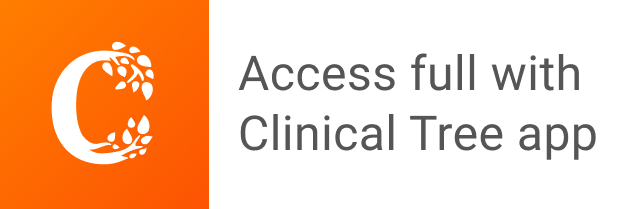