3 Fibroblasts, the majority of cells in ordinary connective tissue, arise from the relevant undifferentiated mesenchymal stem cells1 and are involved in the production of fibrous elements and non-fibrous ground substance (Fig. 3.1). During wound repair they are particularly active and migrate along strands of fibrin by amoeboid movements to distribute themselves through the healing area to start repair. Fibroblast activity is influenced by various factors such as the partial pressure of oxygen, levels of steroid hormones, nutrition and the mechanical stress present in the tissue.2 The other cell types are migrant cells and only occasionally present, such as: macrophages, lymphocytes, mast cells, and granulocutes (Table 3.1).3,4 The extracellular matrix is composed of insoluble protein fibres, the fibrillar matrix and a mixture of macromolecules, the interfibrillar matrix. The latter consists of adhesive glycoproteins and soluble complexes composed of carbohydrate polymers linked to protein molecules (proteoglycans and glycosaminoglycans), which bind water. The extracellular matrix distributes the mechanical stresses on tissues and also provides the structural environment of the cells embedded in it, forming a framework to which they adhere and on which they can move.5 The interfibrillar ground substance is composed of proteoglycans (a family of macromolecules) which bind a high proportion of water (60–70%) and glycoproteins. The latter have a complex shape and are soluble polysaccharide molecules (glycosaminoglycans) bound to a central protein core. In cartilage, the proteoglycans are in turn bound to hyaluronan (a long chain of non-sulphated disaccharides) to form a proteoglycan aggregate – a bottlebrush three-dimensional structure (Fig. 3.2).6 Glycoprotein secures the link between proteoglycan and hyaluronan and also binds the components of ground substance and cells. The fibrous elements are collagen and elastin – both insoluble macromolecular proteins. Collagen is the main structural protein of the body with an organization and type that varies from tissue to tissue. Collagen fibres are commonest in ordinary connective tissue such as fascia, ligament and tendon. The fibrillar forms have great tensile strength but are relatively inelastic and inextensible. By contrast elastin can be extended to 150% of its original length before it ruptures. Elastin fibres return a tissue to its relaxed state after stretch or other considerable deformation. They lose elasticity with age when they tend to calcify. Box 3.1 outlines the components of connective tissue. The basic molecule of collagen is procollagen, synthesized in the fibroblast, illustrated in Figure 3.3, steps 1–4. It is formed of three polypeptide chains (α-chains). Each chain is characterized by repeating sequences of three amino acids – glycine, proline and lysine joined together in a triple helix. The helical molecules are secreted into the extracellular space where they slowly polymerize and crosslink (Fig. 3.4). They overlap each other by a quarter of their length, lie parallel in rows and are collected into large insoluble fibrils. The fibrils unite to form fibres, finally making up a bundle. An aggregate of bundles makes up a whole structure such as a ligament or a tendon. The individual bundles are in coils, which increases their structural stability and resilience, and permits a small physiological deformation before placing the tissue under stress, and in consequence permits a more supple transfer of tractive power in the structure itself and at points of insertion (Fig. 3.5). The process of collagen synthesis is stimulated by some hormones (thyroxine, growth hormone and testosterone), although corticosteroids reduce activity. Fig 3.5 (a) Unloaded collagen fibres in a human knee ligament. (b) Physiological deformation after stress. From Kennedy et al7 with permission (http://jbjs.org/). • Type I: the most abundant of all collagen. Strong thick fibres packed together in high density. It predominates in bone, tendon, ligament, joint capsule and the annulus fibrosus of the intervertebral disc. • Type II: thin fibres found in articular cartilage and the nucleus pulposus of the intervertebral disc. They particularly function in association with a high level of hyaluronan and sulphated proteoglycans to provide a hydrated and pressure-resistant core. • Type III: essentially present in the initial stages of wound healing and scar tissue formation. It secures early mechanical strength of the newly synthesized matrix. These relatively thin, weak fibres are replaced by the strong type I fibres as healing proceeds. Highly fibrous tissues such as ligaments, tendons, fascia and aponeuroses are predominantly collagenous and show a dense and regular orientation of the fibres with respect to each other. The direction of the fibres is related to the stress they experience. Collagen bundles in ligaments and tendons are very strong and rupture usually takes place at the bony attachments rather than by tearing within their substance (Fig. 3.6). Structural and physiological studies8,9 have shown the presence of at least four types of receptor. Three of these have encapsulated endings but the fourth consists of free unencapsulated endings: • Type 1 (Ruffini endings) are present in the superficial layers of a fibrous joint capsule. They respond to stretch and pressure within the capsule and are slow adapting with a low threshold. They signal joint position and movement. • Type 2 are particularly located in the deep layers of the fibrous capsule. They respond to rapid movement, pressure change and vibration but adapt quickly. They have a low threshold and are inactive when the joint is at rest. • Type 3 are found in ligaments. They transmit information on ligamentous tension so as to prevent excessive stress. Their threshold is relatively high and they adapt slowly. They are not active at rest. • Type 4 are free unencapsulated nociceptor terminals which ramify within the fibrous capsule, around adjacent fat pads and blood vessels. They are thought to sense excessive joint movements and also to signal pain. They have a high threshold and are slow adapting. Synovial membrane is relatively insensitive to pain because of the absence of these nerve endings. The mechanical response of a ligament to a load can be represented on a load–deformation curve (Fig. 3.8). In the first part of such a curve (its foot) the ground substance is almost completely responsible for absorbing the stress and displaces the fibres in the direction of the stress. When the load is increased, ligamentous tissue responds slowly and maximum resistance to distraction is only possible if there is enough time for realignment of the collagen bundle. The linear part of the curve shows the slow elastic stretching of the collagen. During this stage, recovery of the original shape of the tissue occurs when the deforming load is removed. This slow rate of deformation is known as ‘creep deformation’. Even in this linear part of the curve, breaking of intermolecular crosslinks begins. For this reason it is assumed that, in physiological circumstances, the load on ligaments is kept within that shown in the foot of the curve, where collagen is not yet under undue strain and the role of ground substance is maximal.10 The composition and the amount of gel ground substance are therefore important in load bearing. On reaching the yield point, a non-elastic or plastic deformation occurs and the ligament progressively ruptures. Some investigators have found that in bone–ligament–bone preparations, separation occurs at the point of insertion.11 Fig 3.8 Mechanical response of the anterior cruciate ligament of the knee to a load. 1. Foot of the curve, the ground substance alone almost completely absorbs the stress. 2. Linear part of the curve, slow elastic stretching of the collagen which is known as ‘creep deformation’. 3. Yield point, a non-elastic or plastic deformation occurs. 4 and 5, the ligament progressively ruptures. Redrawn from Frankel33 with permission. The synovial membrane lines the non-articular parts of synovial joints such as the fibrous capsule and the intra-articular ligaments and tendons within the margins of articular cartilage. The internal surface of the membrane has a few small synovial villi which increase in size and number with age. It also has flexible folds, fringes and fat pads. These accommodate to movement so as to occupy potential spaces and may promote the distribution of synovial fluid over the joint surfaces (Fig. 3.9). Structurally the membrane consists of a cellular intima which is one to four cells deep that rests upon a loose connective tissue subintima and contains the vascular and lymphatic network which has an important function in the supply and removal of fluid. On ultrastructural examination, two cell types (A and B) are apparent. These are closely involved not only with the production of synovial fluid12 but also in the absorption and removal of debris from the joint cavity. The A cells especially have marked phagocytic potential.13 Some synovial cells can also stimulate the immune response by presenting antigens to lymphocytes if foreign material threatens the joint cavity.14 Synovial fluid is a clear, viscid (glairy) substance formed as a dialysate containing some protein. It occurs not only in synovial joints but also in bursae and tendon sheaths. Secretion and absorption are functions of the cells of the intima and of the vascular and lymphatic plexus in the subintima. The synovial initima cells also secrete hyaluronan molecules into the fluid and much evidence has accumulated to show that the viscoelastic and plastic properties of the fluid are largely determined by its hyaluronan content. Chains of hyaluronan bind proteins; these complexes are negatively charged and in turn bind water. The biophysical process is similar to that of the proteoglycans in the matrix of connective tissue and a thick viscous liquid which resembles egg white is formed. Its viscosity varies widely according to circumstances. With a low rate of shear, water is driven out of the hyaluronan–protein complexes and the fluid becomes highly viscous; increase in shear lowers viscosity and the fluid tends to behave more like water. In contrast to viscosity, elasticity increases with higher rates of shear. Both viscosity and elasticity decrease with increasing pH and temperature.15 Articular cartilage is essentially a specialized type of connective tissue. Chondrocytes change with increasing depth from the surface1,16 (Fig. 3.10). In the superficial stratum (zone 1), cells are small, flattened and disposed parallel to the surface. They are surrounded by fine tangentially arranged collagen fibres. A thin superficial layer of this zone has been shown to be cell-free. Cell metabolism in this part is low, which is consistent with the absence of wear and tear in normal healthy tissue. The cells of the intermediate stratum (zone 2) are larger and more rounded and those in the radiate stratum (zone 3) are large, rounded and arranged in columns perpendicular to the surface. In these deeper zones, cells are screened from the coarse fibres by a coat of pericellular matrix bordered by a network of fine collagen fibres. In this way, cells are protected against the stresses generated by load conditions. In the intermediate stratum, collagen fibres are coarser and more spread out to pursue an oblique course that forms a three-dimensional network. In non-load conditions the fibres are orientated at random but when load is applied they are immediately stretched in a direction perpendicular to that of the applied force (Fig. 3.11c). When the load is removed the fibres return to their original oblique position. This behaviour partly explains the resilience and elasticity of cartilagenous tissue. Articular cartilage lacks a nerve supply and is also completely avascular. Nutrition is derived from three sources: synovial fluid, vessels of the synovial membrane and vessels in the underlying marrow cavity which penetrate the deepest part of the cartilage over a short distance. This last source is available only during growth because, after growth is completed, the matrix at the deepest part of the cartilage becomes impregnated with hydroxyapatite crystals which form a zone of calcified cartilage impenetrable by blood or lymph vessels (Fig. 3.10, zone 4). Peripheral nerves also possess supporting connective tissue. Within the nerve trunk the efferent and afferent axons are grouped together in a number of fasciculi (Fig. 3.12). The bundled axons in the fasciculi lie roughly parallel, surrounded by loose delicate collagen fibres running longitudinally along them. Both structures show a wavy appearance which disappears when gentle traction is applied. Each fasciculus is surrounded by a fibrous perineurium, a regular structure of flattened laminae of fibroblasts alternating with fine collagen, running in various directions. These fibroblasts are connected together and form a diffusion barrier against noxious chemical products, bacteria and viruses. In this way, the enclosed axons are to some extent isolated from the external environment. Inside this perineural tube a protein-poor liquid flows centrifugally. This axoplasma is cerebrospinal fluid, which is re-assimilated into the blood circulation at the end of the peripheral nerve. In this respect, the spinal canal and the endoneural spaces are continuous (Fig. 3.13). The epineurium encases the nerve trunk as a collagen coat with little regular organization. Connective tissue surrounding nerves serves as an important mechanical protection to maintain the conductile properties of the nerve.17 During movement, nerves are potentially exposed to tensile forces that can be avoided by mobility in relation to surrounding structures. Here, the wavy form of both axons and surrounding collagen fibres is an important consideration: this ‘waviness’ of the axons is paramount, allowing them to remain relaxed even when the collagen fibres are stretched. Thus, within the normal range of movement, the axons will be protected by the tensile force of the collagen component. When there is a severe sprain or fracture perhaps with dislocation, the range of plastic deformation of collagen can be exceeded and ultimately rupture of collagen fibres and neurotmesis results. The tolerance of nerves to tension is much greater than it is to compression. However, the mobility of nerves allows them to move laterally, so avoiding a compressive force. When space is inadequate for such movement or the nerve is firmly anchored – which is the case in cervical nerve roots – the epineurium may absorb a certain amount of pressure but sooner or later the blood supply within the nerve is affected by increasing compression. Further compression may result in interference with the conductile properties of the nerve. In such circumstances, the Schwann cells and subsequently the myelinated sheath are damaged. Although the axons remain intact, action potentials become blocked, leading to loss of sensory and motor function (see Ch. 2). The muscle cell or myofibril consists of sarcomeres or myofilaments – the basic contractile units of a muscle – arranged in parallel (Fig. 3.14). In each sarcomere two types of filament are distinguishable, chemically characterized as actin and myosin. The actin filaments are each attached at one end to the inner side of the cell membrane forming the so-called Z-line. At the other end they are free and interdigitate with the central myosin filaments. During muscle contraction, the actin filaments slide in relation to the myosin towards the centre of the sarcomere which brings the attachments at the Z-lines closer together with shortening of the whole contractile unit (Fig. 3.15). Finally, the whole muscle is surrounded by the stout epimysium, which is continuous with the septa of the outer perimysium and blends with the connective tissue that forms the tendon, fascia or aponeurosis (Fig. 3.16). These structures are largely composed of collagen fibres with a low amount of proteoglycans. On a dry weight basis, collagen represents 60–80% of the total weight of tendon.18 At the surface, the epitendineum or tendon sheath consists of irregularly arranged condensed collagen as well as elastin fibres. It is continuous with the loosely arranged connective tissue that permeates the tendon between its fascicles and provides a route of ingress and egress for vessels and nerves. At the insertion, the collagen bundles of the tendon permeate into bone. It has been shown19,20,21 that the insertion of the connective tissue of ligaments into bone involves a transition from non-mineralized through mineralized fibrocartilage to bone. In young growing tendons, fibril diameter and tensile strength can be increased by exercise. In adults, however, the effect is minimal although regularly applied tension is necessary to maintain structural integrity. Immobilization has demonstrated loss of tensile strength (see p. 46). Where tendons pass under ligaments or through osteofibrous tunnels, synovial sheaths are formed which separate the tendon completely from its surroundings. These synovial sheaths have two concentric layers, separated by a thin film of synovial fluid and form a closed double-walled cylinder (Fig. 3.17). The fluid acts as a lubricant and ensures easy mobility of the tendon. The internal (visceral) layer is attached to the tendon and the external (parietal) layer to neighbouring structures such as periosteum and retinaculum. Soft tissue injury involves damage to the structural elements of connective tissue with rupture of arterioles and venules. A general inflammatory reaction follows (Table 3.2), one role of which is defensive in that it prompts the subject to restrict activities while recovery takes place. Regardless of the site of injury and the degree of damage, healing comprises three main phases: inflammation, proliferation (granulation) and remodelling. These events do not occur separately but form a continuum of cell, matrix and vascular changes that begin with the release of inflammatory mediators and end with the remodelling of the repaired tissue. Connective tissue regenerates largely as a consequence of the action of inflammatory cells, vascular and lymphatic endothelial cells and of fibroblasts.22,23
Connective tissue
Structural composition
Connective tissue cells
Extracellular matrix (ECM)
Non-fibrous ground substance
Fibrous elements
Regular types
Innervation
Structures containing connective tissue
Synovial joints (Fig. 3.7)
Fibrous capsule and ligaments
Synovial membrane and fluid
Cartilage
Composition
Cartilage cells or chondrocytes occupy small spaces in the matrix
The collagen fibres vary in structure and position with increasing depth from the surface (Fig. 3.11a)
Characteristics of cartilage
Nerves
Muscles
Tendons
Trauma to soft connective tissue
Introduction
Connective tissue
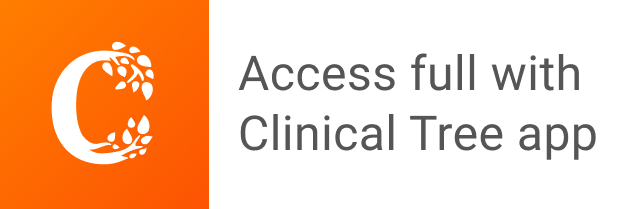