Clinical Assessment of Gait
Olfat Mohamed, Dana Craig, Heather Worden and Edmond Ayyappa
Learning Objectives
On completion of this chapter, the reader will be able to:
1. Describe the major functional tasks of the gait cycle and their corresponding subphases.
3. Define the time and distance parameters used to describe and assess normal gait.
Why do we analyze gait?
The first attempts to analyze gait, recorded in the Rig Veda more than 3500 years ago, most likely was an attempt to enhance mobility through early orthotic or prosthetic intervention. This classic prose chronicles the story of Vispala, a fierce female warrior whose leg, lost in battle, was replaced by an iron prosthesis that enabled her return to the front to fight again.1
Gait assessment describes the patterns of movement that control the progression of the body in walking. Bipedal gait requires a combination of automatic and volitional postural components. This can result in either asymmetric reciprocal movements of the lower limbs (seen in walking or running) or symmetric, simultaneous two-legged hopping. Kangaroos are bipeds that are successful two-legged hoppers.2 Homo sapiens have reached the zenith of movement efficiency in bipedal walking and running by using reciprocal patterns of motion.
Walking requires numerous physiological systems to work congruently. Normal walking requires stability to provide body weight support against gravity during stance, mobility of body segments, and motor control to sequence multiple segments while transferring body weight from one limb to the other. The primary goal in gait is energy efficiency in forward progression by using a stable kinetic chain of joints and limb segments working congruently to transport its passenger unit, consisting of the head, arms, and trunk (HAT) in a continuously changing environment and task demands.
Understanding the process of walking is essential to critically evaluate examination findings and suggest effective intervention plans to help patients improve their walking ability. Clinical gait assessment identifies primary or pathological gait problems and helps differentiate them from compensatory strategies. It is necessary for selection of appropriate orthotic or prosthetic components, alignment parameters, and identification of other variants that might enhance an individual’s ability to walk. Clinical gait assessment also contributes to the development of a comprehensive treatment plan, with the ultimate goal of optimal energy efficiency and appropriate pathomechanical control, balancing cosmesis and overall function.
Kinematic descriptors of human walking
Step length, stride length, cadence, and velocity are important quantitative, interrelated kinematic measures of gait. Step length and stride length are not synonymous. Step length is the distance from the floor-contact point of one (ipsilateral, originating) foot in early stance to the floor-contact point of the opposite (contralateral) foot: in normal individuals the distance from right heel contact to left heel contact. Stride length is the distance from floor contact on one side to the next floor contact on that same side: the distance from right heel contact to the next right heel contact. A reduction in functional joint motion or the presence of pain or muscle weakness can result in decreased stride or step length, or both. Pathological gait commonly produces asymmetries in step length between the two lower limbs.
Cadence is the number of steps taken in a given unit of time, most often expressed in steps per minute. Velocity is the distance traveled in a given unit of time (the rate of forward progression) and is usually expressed in centimeters per second or meters per minute. Velocity is the best single index of walking ability. Decreased joint motion, pain and/or muscle weakness can reduce cadence or velocity or both. Velocity can also be qualitatively categorized as free, slow, or fast. Free walking (self-selected) speed is an individual’s normal self-selected (comfortable) walking velocity. Fast walking speed describes the maximum velocity possible for a given individual while being safe. Slow walking speed describes a velocity below the normal self-selected walking speed. For healthy individuals, a fast walk velocity may be as much as 44% faster than free or self-selected walking speed.3 In people with musculoskeletal and neuromuscular impairments that affect gait, often much less difference is found between free and fast gait velocity.
Double limb support is the period of time when both feet are in contact with the ground. It occurs twice during the gait cycle, at the beginning and the end of each stance phase. As velocity increases, double limb support time decreases. When running, the individual has rapid forward movement with little or no period of double limb support. Individuals with slow walking speeds spend more of the gait cycle in double support.
Step width, or width of the walking base, typically measures between 5 and 10 cm from the heel center of one foot to the heel center of the other foot.4 A wide walking base may increase stability but also reduces energy efficiency of gait.
Ground reaction force (GRF) vector is the mean load-bearing line, which takes into account the forces acting in all three planes. It has magnitude as well as directional qualities. The spatial relation between this line and a given joint center influences the direction of its rotation. The rotational potential of the forces that act on a joint is called a torque or moment.
Gait cycle
A variety of conceptual approaches describe the walking process. Saunders and colleagues5 and Inman and colleagues5 define the functional task of walking as translation of the center of gravity through space in a manner that requires the least energy expenditure. They identify six determinants, or variables, that affect energy expenditure in sustained walking: pelvic rotation, pelvic tilt, knee flexion in stance phase, foot interaction with the knee, ankle interaction with the knee, and lateral pelvic displacement. Individually and collectively, these determinants have an impact on energy expenditure and the mechanics of walking. Although they help us understand the process of walking, the determinants do not themselves offer a practical clinical solution to address the problems of gait assessment.
A comprehensive system to describe normal and abnormal gait has been developed by the Pathokinesiology and Physical Therapy Departments at Rancho Los Amigos Medical Center over the past several decades.4,7,8 The Rancho Los Amigos system serves as the descriptive medium for this chapter (Figure 5-1). Because velocity affects many parameters of walking, the description of normal gait assumes a comfortable self-selected velocity. At free walking velocity, the individual naturally recruits strategies and assumes the speed that provides maximum energy efficiency for their physiological system.
The gait cycle is the period between any two identical events in the walking cycle. Initial contact is traditionally selected as the starting and completing event of a single cycle of gait. Each cycle is divided into two periods: stance phase and swing phase. Stance is the time when the foot is in contact with the ground; it constitutes approximately 60% of the gait cycle. Swing denotes the time when the foot is in the air; it constitutes the remaining 40% of the gait cycle. There are five subphases within the stance period: initial contact (IC), loading response (LR), midstance (MSt), terminal stance (TSt), and preswing (PSw). Swing phase is divided into three subphases: initial swing (ISw), midswing (MSw), and terminal swing (TSw). Because PSw prepares the limb for swing advancement, many consider PSw to be a preparatory component of swing phase.
Three functional tasks are achieved during these eight gait phases: weight acceptance in early stance, single limb support in mid-to terminal stance, and limb advancement during swing.
Functional Task 1: Weight Acceptance
IC and LR are the subphases of stance where weight acceptance is accomplished. Effective transfer of body weight onto the limb as soon as it makes contact with the ground requires initial limb stability, shock absorption, and the preservation of forward momentum.
Initial Contact
IC is the instant that the foot of the leading lower limb touches the ground. Most motor function during IC is preparation for LR. At IC, the ankle is in neutral position, the knee is close to full extension, and the hip is flexed 30 degrees. The sagittal plane GRF vector lies posterior to the ankle joint, creating a plantar flexion moment (Figure 5-2). Eccentric contraction of the pretibial muscles (tibialis anterior and long toe extensors) holds the ankle and subtalar joint in neutral position. At the knee, the GRF vector is anterior to the joint axis, which creates a passive extensor torque. Muscle contraction activity of the three vasti of the quadriceps and hamstring muscle groups continues from the previous TSw to preserve the neutral position of the knee joint. A flexion moment is present around the hip joint because the GRF vector falls anterior to the joint axis. Gluteus maximus and hamstring muscles are activated to restrain the resultant flexion torque.
Loading Response
LR occupies approximately 10% of the gait cycle and constitutes the period of initial double limb support (see Figure 5-2B). Two functional tasks occur during LR: controlled descent of the foot toward the ground and shock absorption as weight is transferred onto the stance limb.
The momentum generated by the fall of body weight onto the stance limb is preserved by the heel rocker (first rocker) of stance phase. Normal IC at the calcaneal tuberosity creates a fulcrum about which the foot and tibia move. The bony segment between this fulcrum and the center of the ankle rolls toward the ground as body weight is loaded onto the stance foot, preserving the momentum necessary for forward progression. Eccentric action of the pretibial muscles regulates the rate of ankle plantar flexion, and the quadriceps vasti contract to limit knee flexion. The action of these two muscle groups provides controlled forward advancement of the lower extremity unit (foot, tibia, and femur). During the peak of LR, the magnitude of the vertical GRF exceeds body weight. To absorb the impact force of body weight and preserve forward momentum, the knee flexes 15 to 18 degrees and the ankle plantar flexes to 10 degrees. The hip maintains its position of 30 degrees of flexion. Contraction of the gluteus maximus, hamstrings, and adductor magnus prevents further flexion of the hip joint.
Functional Task 2: Single Limb Support
Two phases are associated with single limb support: MSt and TSt. During this period, the contralateral foot is in swing phase, and body weight is entirely supported on the stance limb. Forward progression of body weight over the stationary foot while maintaining stability must be accomplished during these two subphases of stance.
Midstance
MSt begins when the contralateral foot leaves the ground and continues as body weight travels along the length of the stance foot until it is aligned over the forefoot at approximately 20% of the gait cycle (Figure 5-3). This pivotal action of the ankle rocker (second rocker) advances the tibia over the stationary foot. Forward movement of the tibia over the foot is controlled by the eccentric contraction of the soleus assisted by the gastrocnemius.
During this phase, the ankle moves from its LR position of 10 degrees of plantar flexion to approximately 5 degrees of dorsiflexion. The knee extends from 15 degrees of flexion to a neutral position. The hip joint moves toward extension, from 30 to 10 degrees of flexion. With continued forward progression, the body weight vector moves anterior to the ankle, creating a dorsiflexion moment. Eccentric action of the plantar flexors is crucial in providing limb stability as contralateral toe-off occurs, transferring body weight onto the stance foot. By the end of MSt, the body weight vector moves anterior to the knee (creating passive extensor stability at the knee) and posterior to the hip (reducing the demand on the hip extensors). The gluteus maximus, active in early MSt, ceases its activity and now stability relies on passive structures as the hip nears vertical alignment over the femur. Vertical GRF is reduced in magnitude at MSt because of the upward momentum of the contralateral swing limb. In the coronal plane, activity of hip abductors during MSt is essential to provide lateral hip stability and almost a level pelvis.
Terminal Stance
TSt, the second half of single limb support, begins with heel rise of the stance limb and ends when the contralateral foot makes contact with the ground. As the body vector approaches the metatarsophalangeal joint, the heel rises and the phalanx dorsiflexes (extends). The metatarsal heads serve as an axis of rotation for body weight advancement (see Figure 5-3B). This is referred to as the forefoot or toe rocker (third rocker). The forefoot rocker serves as an axis around which progression of the body vector advances beyond the area of foot support, creating the highest demand on calf muscles (gastrocnemius and soleus). During TSt, the ankle continues to dorsiflex to 10 degrees. The knee is fully extended, and the hip moves into slight hyperextension. Forward fall of the body moves the vector further anterior to the ankle, creating a large dorsiflexion moment. Stability of the tibia on the ankle is provided by the eccentric action of the gastrocsoleus muscles.
The trailing posture of the limb and the presence of the vector anterior to the knee and posterior to the hip provide passive stability at hip and knee joints. The tensor fascia lata serves to restrain the posterior vector at the hip. At the end of TSt, the vertical GRF reaches a second peak greater than body weight, similar to that which occurred at the end of LR.
Functional Task 3: Limb Advancement
Four phases contribute to limb advancement: PSw, ISw, MSw, and TSw. During these phases the stance limb leaves the ground, advances forward, and prepares for the next IC.
Preswing
PSw, the second period of double limb support in gait, comprises the last 10% of the stance phase. It begins when the contralateral foot makes contact with the ground and ends with ipsilateral toe-off. During this period, the stance limb is unloaded and body weight is transferred onto the contralateral limb (Figure 5-4). The ankle moves rapidly from its TSt dorsiflexion into 20 degrees of plantar flexion. During this subphase, plantar flexor muscle activity decreases as the limb is unloaded. Toward the end of PSw, the vertical force is diminished such that plantar flexors rapidly decrease their activity to complete quiescence. There is no active muscle contraction for “push off” in normal reciprocal free walk bipedal gait.6 The knee also flexes rapidly to achieve 35 to 40 degrees of flexion by the end of PSw. The GRF vector is at the metatarsophalangeal joints and posterior to the knee, creating passive knee flexion with toe clearance. Knee flexion during this phase prepares the limb for toe clearance in the swing phase. PSw hip flexion is initiated by the rectus femoris and the adductor longus, which also decelerates the passive abduction created by contralateral body weight transfer. The sagittal vector extends through the hip as the hip returns to a neutral position.
Initial Swing
Approximately one third of the swing period is spent in ISw. It begins the moment the foot leaves the ground and continues until maximal knee flexion (60 degrees) occurs, when the swinging extremity is directly under the body (see Figure 5-4B). Concentric contraction of pretibial muscles initiates foot dorsiflexion from its initial 20 degrees to 5 degrees of plantar flexion. This is necessary for toe and foot clearance as swing phase begins. Knee flexion, resulting from action of the short head of the biceps femoris, also assists in toe clearance. The knee continues to flex until it reaches a position of 60 degrees of flexion. Contraction of the iliacus advances the hip to 20 degrees of flexion. Contraction of the gracilis and sartorius muscles during this phase assists hip and knee flexion.
Midswing
During MSw, limb advancement and foot clearance continue. MSw begins at maximum knee flexion and ends when the tibia is vertical. Knee extension, coupled with ankle dorsiflexion, contributes to foot clearance while advancing the tibia (see Figure 5-4C). Continued concentric activity of pretibial muscles ensures foot clearance and moves the foot toward the neutral position. Momentum creates an extension moment, advancing the lower leg toward extension from 60 degrees to 30 degrees of flexion, with the quadriceps quiescent. Mild contraction of hip flexors continues to preserve the hip flexion position.
Terminal Swing
In the final phase, TSw, the knee extends fully in preparation for heel contact (see Figure 5-4D). Eccentric contraction of the hamstrings and gluteus maximus decelerates the thigh and restrains further hip flexion. Activity of the pretibial muscles maintains the ankle at neutral to prepare for heel contact. In the second half of TSw, the rectus femoris is quiescent but the rest of the quadriceps vasti become active to facilitate full knee extension. Hip flexion remains at 30 degrees.
Describing pathological gait
Clinicians often use qualitative descriptive terms to characterize gait deviations and compensations. Some of these terms help identify specific primary problems; others describe compensatory strategies used by patients to solve gait difficulties created by various primary impairments.
Common Gait Deviations Observed During Stance
Trendelenburg gait occurs in the stance phase, when the trunk leans to the same side as the hip pathology (ipsilateral lean). This is a compensatory strategy used when the gluteus medius muscle and its synergists (gluteus minimus and tensor fascia lata) cannot adequately stabilize the pelvis during stance.9 Normally the drop of the contralateral pelvis is limited to 5 degrees by the eccentric control of the strong hip abductor muscles. To support the pelvis, the hip abductor muscles must generate a force that is 1.5 times the body weight.10 Weak or absent gluteus medius muscle leads to a postural substitution of an ipsilateral trunk lean over the weight-bearing hip joint. This reduces the external adductor moment created by a GRF line that falls medial to the joint center. Without this postural compensation, clearance becomes a problem for the swinging contralateral limb. Rarely, Trendelenburg gait is caused by overactive hip adductors (adductors longus, magnus, brevis, and gracilis).
Vaulting is exaggerated heel elevation of the stance foot, occasionally occurring with simultaneous stance limb hip and knee extension, with the goal of raising the pelvis to clear the contralateral swing limb. It occurs when the functional length of the swing limb is relatively longer than that of the stance limb. It also occurs when swing limb advancement is impaired or delayed by inadequate motor control of hip or knee flexion, or both, or in the presence of a plantar flexion contracture of the swing leg. It may compensate for pelvic obliquity or leg length discrepancy.
Antalgic gait is a strategy used to avoid pain during walking. It is frequently observed in LR when the patient reduces single limb support time on the affected limb. If the pain occurs during a particular interval in stance, that time interval is avoided. Antalgic gait caused by pain that originates around the hip might translate into a lateral lean to permit the patient to get the center of gravity over the support point, the head of the femur. If the pain occurs during the extreme end range of a particular joint motion, that motion is diminished. For example, if full extension produces pain, the knee would be maintained in slight flexion throughout the gait cycle.
Common Gait Deviations Observed During Swing
Circumducted gait is a swing phase deviation in which hip abduction is combined with a wide arc of pelvic rotation, most often occurring as a compensatory pattern when there is a relatively longer swing limb compared with the stance limb. This action is most likely to happen when there is limited motion or impaired motor control affecting ankle dorsiflexion, knee flexion, or hip flexion. A plantar flexion contracture at the foot or a stiff knee or hip joint can necessitate a circumduction pattern during swing in an effort to achieve toe and foot clearance. This combination of abduction and pelvic rotation is a compensatory strategy to advance the limb.
Circumduction can be observed as a lateral arc of the foot in the transverse plane that begins at the end of PSw and ends at IC on the same limb. The arc reaches the apex of its lateral movement at MSw. The typical pattern is a mixture of a wide base of support with the foot abnormally outset and may include an ipsilateral pelvic drop. It is possible for a contracture of the contralateral adductors to create this deviation by pulling the pelvis toward the contralateral femur and demanding a compensatory ipsilateral abducted position relative to the pelvis. A severe leg length discrepancy can result in an exaggerated pelvic tilt from the contralateral stance leg, which obligates the swing limb to an increased abduction position. Circumduction and abduction create a significant energy cost penalty, increasing lateral displacement of the center of gravity.
Gait Deviations Associated with Abnormal Muscle Tone
A variety of abnormal gait patterns are associated with abnormal muscle tone (spasticity, rigidity, hypotonicity, or abnormal motor control) or muscle weakness. Scissors gait describes a pattern of poor control in limb advancement or tracking of the swing leg often characterized by the crossing, or scissoring (hip adduction, flexion and medial rotation), of the lower limbs. It is most often observed in patients with spastic or paretic pathological conditions such as hemiplegia, spastic diplegia, and cerebral palsy. Steppage gait occurs when there is weakness or paralysis of the dorsiflexor musculature, such as in persons with peroneal palsy or peripheral neuropathy, demands exaggerated hip and knee flexion of the proximal joints to accomplish swing clearance. It is most easily observed in late MSw.
In crouch gait exaggerated knee and hip flexion occurs throughout the gait cycle. Crouch gait is often seen in combination with a toe-walking stance in children and adults with spastic diplegic cerebral palsy. It has been attributed to a combination of overactivity of the hamstrings and weakness of calf muscles. Although orthosis use can successfully control abnormal motion in the sagittal plane (e.g., in steppage gait), orthoses are less effective in controlling the abnormal transverse, rotational, or coronal limb placement problems observed in scissoring or crouched gait pattern.
In ataxic gait there is a failure of coordination or irregularity of muscular action of the limb segments commonly caused by cerebellar dysfunction. Ataxia often becomes accentuated when the eyes are closed or vision is impaired or distracted.
Qualitative gait assessment
Qualitative methods for identification and recording of gait deviations have played a role in patient care for decades. In 1925, Robinson11 described pathological gait patterns and attempted to correlate them with specific disease processes. In 1937, Boorstein12 identified 14 disease processes that could be diagnosed with gait assessment. He described seven major gait deficit groups, attributing the term steppage gait to the French physician Charcot and the identification of waddling gait in hip dysplasia to Hippocrates. In the late 1950s, Blair Hangar, the founder of Northwestern University’s School of Prosthetics and Orthotics, and Hildegard Myers, a physical therapist at Rehabilitation Institute of Chicago, collaborated to develop the first comprehensive system of clinical gait analysis for persons with transfemoral amputation.13 They identified 16 gait deviations and suggested numerous clinical and prosthetic causes for each. Their work, developed into an educational film and handbook in 1960, has been a model for subsequent instructional videos and assessment systems in prosthetics.14 Brunnstrom’s15 comprehensive gait analysis form for hemiplegic gait, published in 1970, is a checklist of 28 deviations seen at the ankle, knee, and hip that are common after stroke.
Early work in observational gait analysis received a significant impetus from Perry16 as an outgrowth of basic research data published in 1967. In the late 1960s, Perry and a group of physical therapists from the Rancho Los Amigos Medical Center Physical Therapy Department developed an organized format for systematically applied observational gait analysis. Their work initially focused on the development of an in-house training program for students and personnel who were new to the rehabilitation hospital. The first Normal and Pathological Gait Syllabus was published by the Professional Staff Association of Rancho Los Amigos Hospital in 1977.7 Subsequent revisions have included additional gait data and gait interpretation.17 This syllabus uses parameters of normal gait as a comparative standard for abnormal or pathological gait. It focuses on identifying gait deviations that affect the three functional tasks of walking: weight acceptance, single limb support, and swing limb advancement. A form listing the most commonly occurring gait deviations in each subphase of gait is used to record any observed gait deviations that interfere with these functional tasks (Figure 5-5). Problems in each of the six major body segments are noted with a check in one of the boxes, beginning with the toes, then the ankle, knee, hip, pelvis, and trunk. This format allows the clinician to consider systematically the following questions:
• Are the toes up, inadequately extended, or clawed?
• Does the trunk lean or rotate backward or forward? Does it lean laterally to the right or left?
Qualitative gait assessment is an important component of preorthotic assessment because it assists the clinician in identifying the functional task and the subphase of gait that are problematic and can be addressed with orthotic intervention. Similarly, deviations observed during gait analysis can identify the need for adjustment of prosthetic alignment.
Instrumented gait analysis
Instrumented gait analysis records the process of walking with measurable parameters collected through the use of equipment. Such basic techniques would have enabled measurement of walking velocity (distance traversed per unit of time) and cadence (steps per unit of time). Marks,18 a New York City prosthetist, offered a more precise qualitative description of pathological gait in 1905, when he described the gait process in eight organized phases and discussed the implications of prosthetic component design on walking function. Marks praised “kinetoscopic” photography as a potential diagnostic tool for optimizing pathological gait.
Today we record gait parameters with instruments as common as a stopwatch or as complex as the simultaneous integration of three-dimensional kinematics, kinetics, and electromyographic (EMG) methods. The primary emphasis of clinical assessment has been on accessible techniques and inexpensive technologies. A simple, inexpensive footprint mat has been used for decades to record barefoot plantar pressures. Clinics use individual or multiple mats to record step and stride length as well as walking base width. Early on, video technology with slow-motion capabilities made more precise qualitative description of the gait cycle possible. The continued development of inexpensive video gait assessment software has made clinical quantitative applications more practical as well. Most quantitative and qualitative video systems, however, measure joint angles in two dimensions, which does not offer a complete analysis of the three-dimensional walking activity.
Technology in Gait Assessment
The high-tech side of quantitative gait analysis has traversed a surprisingly long road. The birth of instrumented kinematic, EMG, and temporal performance analysis began in the 1870s with E. J. Marey, who first performed movement analysis of pathological gait with photography.19 He also developed the first myograph for measuring muscle activity and the first foot-switch collection system for measuring gait events related to the temporal parameters. The foot-switch system was an experimental shoe that measured the length and rapidity of the step and the pressure of the foot on the ground. Eadweard Muybridge,20 working at Stanford University in the 1880s, used synchronized multiple camera photography with a scaled backdrop to record on film and assess the motion of subjects walking. Scherb made other major advances in instrumented gait analysis in 1920 by performing manual muscle palpation on individuals while using a treadmill. Additionally, in 1925 Adrian advocated the use of EMG to study the dynamic action of muscles.21
Modern gait technology began in 1945, when Inman and colleagues initiated the systematic collection of gait data for individuals without impairment and with amputation in the outdoor gait laboratory at the University of California at Berkeley.5 Since then, researchers and clinicians have increasingly used the wide array of gait technologies to measure the parameters of human performance in normal and pathological gait. A full-service gait laboratory gathers information on six performance parameters in walking: temporal, metabolic, kinematic, kinetic, EMG, and pressure.22
Measuring Temporal and Distance Parameters
Temporal (time, distance) parameters enable the clinician to summarize the overall quality of a patient’s gait. Temporal data collection systems might be one of the most effective components available for assessment in the clinical setting. In the gait laboratory, microswitch-embedded pads taped to the bottom of a patient’s shoes or feet can record the amount of time that the patient spends on various anatomical landmarks over a measured distance. Portable pressure-sensitive gait mats, connected to a laptop computer with gait analysis software for time and distance parameters are also commercially available to use in clinical settings (Figure 5-6).23,24 For example, the GAITRite system, which consists of an electronic walkway connected to a computer, records the temporal and spatial characteristics of patients while walking as well as while performing other functional or occupational tasks. The GAITRite mat is flexible and can be rolled and transported in a hard case, which enables data collection at different clinics or sites. Wening and colleagues used the GAITRite system to validate the effect of using an ankle-foot orthosis (AFO) on the walking pattern of patients with stroke.25 In this study, they compared stride length, velocity, cadence, percentage of the gait cycle spent in single and double limb support, as well as general stance progression patterns. Gait deviations related to excessive inversion, eversion, or prolonged heel-only time can be recognized and considered when modifying the alignment or components of prostheses or orthoses. A temporal data collection system is particularly cost effective and clinically meaningful. Temporal data are usually a product of another measuring system such as EMG or motion analysis. Temporal data systems are commercially available covering a wide range of cost, technical sophistication, and time required to analyze the summarized data. Some of the temporal parameters, however, can be recorded, to a lesser degree of accuracy using a stopwatch and video camera.26
Assessing the Energy Cost of Walking
Metabolic data reflect the physiological “energy cost” of walking. The traditional measures of energy cost are oxygen consumption, total carbon dioxide generated, and heart rate. Other relevant factors include volume of air breathed and respiratory rate. All these parameters are viewed in relation to velocity and distance walked over the collection period. Historically, metabolic data were collected while the patient walked on a treadmill, wearing umbilical devices. In recent years, because of the known influence of treadmill collection in altering normal gait velocity, energy cost data are more likely to be obtained on an open track of a measured distance with the patient ambulating in a free walk or natural cadence (Figure 5-7). With the cardiopulmonary device market continually growing and advancing, there is a wide array of versatile testing equipment to choose from. This equipment allows the patient to negotiate their normal environments with little or no interruption due to the testing and collection setups. Some of the newest products on the market couple the traditional oxygen and carbon dioxide (VO2 and VCO2) measurement with the capability of collecting telemetry data, indirect calorimetry, and integrated electrocardiogram among other add-ons to standard systems. The primary limitation of energy cost as an assessment tool is that, although it can inform the investigator about body metabolism relative to the patient’s gait, it cannot explain why or how an advantage or disadvantage was obtained. Waters27 demonstrated that an individual with Syme’s level amputation uses less oxygen to traverse a given distance than someone with a transtibial amputation but could not explain why. For that explanation, other gait parameters must be examined. Energy cost measures cannot easily identify widely variant prosthetic foot designs worn by the same patient, whereas kinematic, kinetic, and EMG data typically can.28 The Oxycon Champion respirometry system has been used in many studies; one involved measuring the step-by-step metabolic consumptions of amputees. This system allowed for unrestricted locomotion which made the data collection easier and more relevant to everyday situations of the participant.29 The CPX by Medgraphics, a breath-by-breath analysis system for cardiopulmonary exercise testing, was used to record similar data relating to the biomechanics of lower-limb amputee gait and metabolic data.30
Perhaps the best kept secret in the energy cost arsenal is the physiological cost index (PCI). It is easily calculated as follows:

The PCI is one of the most sensitive indicators of energy cost of gait. Winchester and colleagues31 compared two different orthotic designs by measuring a wide variety of metabolic parameters as well as the PCI. Their results demonstrated statistically significant difference in PCI between the two devices when all other measured parameters failed to produce such differences. Pulse and respiratory rate taken at rest and after timed intervals during normal comfortable gait can also help assess exertion levels.
Kinematic and Kinetic Systems
Most kinematic systems provide joint and body segment motion in graphic form. This information includes sagittal, coronal, and transverse motions that occur at the ankle, knee, hip, and pelvis. The patient is instrumented with reflective spheres that are placed on well-recognized anatomical landmarks (Figure 5-8). Typically, an infrared light source is positioned around each of several cameras. This light is directed to the reflective spheres, which in turn are reflected into the cameras. Each field of video data is digitized, an operator manually identifies the markers, and the coordinates of the geometric center of each marker are calculated with computer software. Resultant data are displayed as animated stick figures that represent the actual motions produced by the patient. The operator can freeze any frame and enlarge the image at any joint to examine gait patterns in greater depth. The operator can extract raw numbers that represent joint placement and motion in space or produce a printout showing joint motion in all planes plotted against the percentage of the gait cycle (Figure 5-9). Angular velocities, accelerations, and joint and segment linear displacements can be calculated. Data from other systems (force platforms and EMG) collected during the same time sequence as the motion data are often integrated with the kinematics. Advanced systems like these can be a very expensive component of the gait lab, but the data collected provides some of the most in-depth and valid data. In the gait lab or a clinical lab, the motion system setup serves as the technological core. A variety of Vicon motion systems have been used to evaluate the joint motion in patients with spastic diplegic cerebral palsy and various other patient populations.32 Similarly the EvaRT motion analysis system has been used to collect data comparing mechanical and microprocessor knees in patients with gait and balance deficits.33
The Dartfish system is another motion analysis tool that is used in gait laboratories and clinical settings.34 The Dartfish system allows for two- and three-dimensional joint motion analysis. It is portable, less expensive, and requires less time to set up when compared with other motion analysis systems. Dartfish has been used to record and immediately evaluate the effects of various prosthetic feet on knee flexion during normal walking. These tools can allow for improved technique and transmission of information to patients and optimally a decrease in recovery time.35
When an individual takes a step, he is exerting force against the surface he is walking on. This kinetic information is obtained from one or more force platforms, which collect data on the three components of the ground reaction force: vertical, fore-aft (anterior-posterior), and medial-lateral (Figure 5-10). The contribution of kinetic data can be significant. Fore-aft shear is quite useful in establishing appropriate transtibial prosthetic alignment in the sagittal plane. For this purpose the clinician would anticipate a balanced magnitude and timing of the braking and propulsive patterns. Data collection from two consecutive steps, one gait cycle, requires dual force plates. Some kinetic software packages also offer specialized programs for specific purposes such as stability analysis, which provides information about center of gravity shift relative to time.
While the typical force platform system provides data about forces and moments occurring at the ground, or center of pressure progression, it can be combined with kinematic data to provide additional information. By combining these two data sets, the moments and power acting at the joints can be calculated. This information is useful in measuring the dynamic joint control of an individual throughout stance, particularly when used in conjunction with EMG. Similarly, information about joint moments, sometimes referred to as torque, is also often reported as an outcome measure in research studies. While this information can be potentially important in the evaluation of pathological gait, it is also necessary to have a basic understanding of how these values are derived. As mentioned, as an individual ambulates, the individual exerts force on the walking surface; differing degrees of this force are similarly exerted on each of the joints in the lower extremity. With the exertion of these forces comes an associated moment that is also acting at the joint, along with a power value. In its most basic form, a moment is the result of a force multiplied by a distance or lever arm.6 Joint power is then calculated by multiplying the moment acting at a joint by the joint’s angular velocity. Additionally, the moment acting on a particular segment is most frequently calculated with reference to the center of mass of that segment. This means that the lever arm is the distance from where the forces are acting at the joint to the center of mass of the segment. In order to calculate these values, the lower extremity must be broken down into segments, often the ankle, shank or calf, and thigh. By doing this, a link-segment model is being applied and the parameters of interest can be calculated.36
To further illustrate, the interrelated nature of these measures, the calculation path for forces, moments, and power is also presented (Figure 5-11). It is important to note within the diagram where the different data sources originate. There are very few directly measured values that are then combined with biomechanical models to calculate these variables.
The calculation process begins with the determination of the ground reaction forces, which are obtained through the direct measurement of an individual stepping on a force platform. Once that information is available, it is combined with kinematic data, derived from a two- or three-dimensional motion capture system for each lower extremity body segment, so that the joint reaction forces can be calculated. As the forces at each of the joints are determined, then the associated moments acting on each segment can also be calculated. Ultimately, the power can be calculated as well (Figure 5-12).
In many cases, instrumented kinetic and kinematic systems have included an inverse dynamics model that is applied to determine the forces acting at each of the lower extremity joints. Like virtually all biomechanics models, certain assumptions must be made in order for the calculation to be carried out in a practical manner. With assumptions come the opportunity for additional error introduction throughout the process. This is why it is important to understand the limitations associated with them. A fundamental point is that frequently these calculations all rely upon data that are calculated using general body proportions and anthropometric models for whole bodied individuals. Because of this, certain assumptions are made about the mechanical properties of the segments and joints being evaluated. For example, many of the commonly used models assume that the subject has no limb deficiencies and essentially normal musculature. While this may be acceptable for evaluations of individuals without pathology, these assumptions can become a source of error when evaluating an individual with an amputation or other limb dysfunction. There is also the issue that the knee and ankle joints are frequently modeled as simple hinge joints. By doing this it makes the calculations more practical to perform but does not completely represent the anatomical reality. Particularly in the case of the knee, the joint center does not stay in a fixed position during stance, but many of the models for calculating joint moments assume that it does (Figure 5-13). As a result, there can be variation in the distance used to calculate the moment at the knee. Considering the physical location, even a small variation in the estimated joint center could result in a significant change in the value calculated. Because many of the calculations rely upon the model assumptions, the inherent errors can be easily compounded. This is not to say that these variables should be ignored but that their value should be tempered with an understanding of the process for obtaining them.
Electromyography
Muscle action beneath skin and subcutaneous tissue cannot be directly measured, but through the use of EMG, the activity can be approximated and studied in relation to the action, size of muscle, and signals obtained. EMG records the muscle activity by the electrical signal detected from the contraction and chemical stimulation of the respective musculature.6 EMG instrumentation can vary, such as is seen with surface EMG or fine-wire EMG. With surface EMG the electrode pad is adhered to the skin above the muscle being studied, while fine-wire EMG uses wire electrodes directly inserted into the belly of the respective muscle. Intrasocket EMG is a relatively new technique employing traditional surface EMG techniques as well as the use of transcutaneous electrical nerve stimulation (TENS) techniques to allow for EMG to be worn by amputees underneath their prosthesis. This technique allows for EMG information to be gathered on amputees during walking and other dynamic activities.37 EMG data may be the single most important technology in terms of understanding the direct physiological effect of gait variants.
EMG records the motor unit activation of muscle fibers in the specific muscle being studied. This is very useful but can be problematic with surface electrode applications, in that they can pick up the signal from surrounding musculature during testing. EMG characterization allows for timing, relative intensity of muscular effort, as well as resultant muscle force, all of which are necessary to understand normal and pathological gait. EMG data are normalized against maximum contraction data for each respective muscle. Without normalization, the data collected may be invalid and can lead to erroneous interpretation. Maximum contraction is dependent on joint angle as well as the duration of the contraction, both of which are influential to the overall information extracted from analysis.
Patterns of muscle activity in patients with abnormal gait are compared with well-established norms. Knowledge of the timing and intensity of the muscle activity throughout the gait cycle may guide gait training, orthotic or prosthetic prescription, and dynamic orthotic or prosthetic alignment aimed at reduction of excessive, ill-timed, or prolonged muscle activity. EMG is exceedingly adaptable with the most basic function of superficial muscle activity data to intramuscular fine wire sensor technology, which is all cohesive to the implementation of many other complex clinical or gait lab technologies.26 EMG data are also helpful in guiding decisions about surgical intervention (dorsal rhizotomy, tendon lengthening, or osteotomy) in children with cerebral palsy.
Pressure-Sensing Technology
Pressure-sensing technologies offer the clinician tremendous insights into the treatment of patients at risk for amputation because of vascular disease and diabetic neuropathy. They can also assist vascular surgeons and orthopedic foot specialists in limb salvage through more appropriate custom-designed prophylactic orthoses. In some systems, a thin plastic array can slip nearly unnoticed between the plantar surface of the foot and an orthosis or insole of the shoe (Figure 5-14). This array, connected to a computer by a lead wire, can measure dynamic pressure patterns and record critical events throughout the walking cycle. A prosthetic version can provide various measurements at 60 individual sites within a socket and record those measurements during multiple events of the gait cycle. Pressure is expressed in terms of a force over the area at which the force is acting.

Cavanaugh and colleagues state that “Pressure measurement offers a way to help the clinician break out of the cycle of trial and error that is so often necessary to find the correct solution to a patient’s problem.”38 Currently surface pressure measurement systems exist in various forms ranging from in-shoe, barefoot, seating and positioning, joint, and prosthetic and orthotic floor-based models. Over the years, Perry and colleagues have collected data on the most common types of pressure testing systems that consist of a “force plate” that uses ink and paper to record the areas of peak pressures during ambulation. This type of system is inexpensive and provides reliable, easily interpreted data. Instrumented insoles, however, are positioned inside the patient’s shoes and worn during ambulation and activity performance. The insoles record pressures and various forces on the plantar surface of the foot through an integrated array of sensors.6 The diversity of treatment applications has promoted these systems to one of the most valuable in the laboratory setting.26 An example of the application of pressure sensing technology is the development of the dynamic gait stability index, which is based on plantar pressure and utilized the F-Scan insole sensors from Tekscan Inc. The compiled data was used to evaluate dynamic gait stability through six different parameters and were then indexed across different walking conditions, terrains, and speed.39–41 This area is one of the more recent and clinically promising technologies for the assessment team.
Choosing the appropriate assessment tool
Over the past several decades, technologies have advanced and aided in providing a significantly improved understanding of pathological gait. They have also assisted clinicians in providing strong evidence for the efficacy of various treatment approaches and ultimately helped enhance patient care. Advocates of a more universal application of the high-end technologies in the clinical setting have made a compelling case for implementation in gait laboratories and clinical settings alike across the field of rehabilitative care. Determining the extent and necessity for various high-end devices, such as full featured motion analysis systems, in the clinical setting can be a very extensive process requiring the evaluation of the advantages and disadvantages to both the patient and the clinic, particularly in the current climate of cost containment. Perhaps the strongest argument for gait technology in our present era lies in its use for outcome measurement to justify legitimate therapeutic treatment approaches as well as orthotic and prosthetic applications.
Recognizing the need, benefits, and drawbacks of technology in the clinical setting is very important. Appropriate instrumented evaluations will need to be made by the rehabilitation team to help optimize patient outcomes.
Function-based assessment
Task-oriented tests or functional measures are performance based tools directly related to specific activities and linked to “real-world” domains of function. For example walking in the community requires meeting the demand of varied distance, terrain, illumination, obstacles, stair-climbing, and multitasking. These tests usually measure performance of the individual against established norms for a specific population. Results of functional measures serve as a baseline to establish goals and benchmarks and evaluate progress after intervention.
Holden and colleagues42 suggest that gait performance goals for patients with neurological impairments are best measured against values from impaired rather than healthy subjects. Treatment goals are adjusted for the individual patient’s diagnosis, etiologic factor, ambulation aid, and functional category. In separate studies, Brandstater and colleagues43 and Holden and colleagues42 found that patients with the greatest number of gait deviations did not have the lowest temporal values. A great deal of energy is often expended by physical therapists, prosthetists, and orthotists in an attempt to help patients achieve optimal gait patterns. Holden and colleagues42 suggest that hard-won qualitative gait improvements may cause secondary losses in time-distance parameters, such as slower velocity and reduced step length. The fundamental issue is whether temporal gait efficiency or cosmesis should be the preferred goal. Certainly, in cases in which patients are nominal walkers and in which therapy, surgery, and orthotics or prosthetics have optimized, gait efficiency is far more important than reducing in compensatory gait deficits.
In the past, symmetry and reciprocal movement patterns have been significant treatment goals. Wall and Ashburn44 maintain that “an ideal objective in the functional rehabilitation of hemiplegia is the reduction of the asymmetrical nature of movement patterns.” Measuring pathological gait against normal gait values is a useful means of providing an overall clinical picture. In setting treatment goals, however, measuring a patient’s performance against their own best possible outcome is more reasonable. How can a given patient’s best possible outcome be anticipated? This requires collection of accurate data to establish pre-treatment and post-treatment profiles for a wide variety of involvement levels within each pathological condition. Olney and Richards45 suggest that large groups of instrumented studies be undertaken to identify clusters of biomechanical features associated with functional performance during walking
Time-distance parameters have enormous potential for setting outcome goals. Variations in time-distance values are often specific to pathological condition. Asymmetries in hemiplegia, for example, are obviously greater than in most other types of pathological conditions. Variables that are reported to affect temporal measurements in normal healthy subjects include age, gender, height, orthotic use, or type of assistive device. In separate studies of patients with pathological conditions, Brandstater and colleagues43 and Holden and colleagues42 found no significant difference in temporal performance based on gender or age.
Corcoran and colleagues46 measured temporal parameters of subjects with hemiplegia under two gait conditions: with and without their AFO. Patients with hemiplegia had significantly faster gait velocity when wearing their orthoses than when walking without them.46 Another similar study of healthy unimpaired subjects wearing AFOs found reduced step length. Apparently subjects without central nervous system involvement altered their movement strategy to decrease movements at the knee in an effort to minimize shearing forces in the AFO.47 Reduced step length can minimize force exerted by the brace along the posterior aspect of the calf band.48
Functional Measures
A wide variety of functional measures are available to provide information about ambulatory function of individuals with neuromuscular, musculoskeletal, cardiopulmonary, or metabolic diseases and conditions. Most of these measures do not require specialized equipment or instrumentation. Some of the principal functional measures currently in use are (1) Functional Ambulation Classification System (FAC); (2) Functional Independence Measure locomotion and mobility subscales (FIM); (3) Gait Abnormality Rating Scale (GARS); (4) Timed Up and Go Test (TUG); and (5) Dynamic Gait Index (DGI). These measures provide information about how gait impairments affect function in the person’s environment, rather than about specific gait problems or deviations. Most of these measures require some degree of practice or training so that testers may develop accuracy in using the tool and can apply all of its psychometric implications.
Walking Speed
Walking speed (WS) (or gait velocity) is the fundamental walking measure which defines the person’s basic walking ability.6 It is the time required for a person to traverse a specific distance. The term velocity indicates not only the speed of travel but also the specific direction of travel. Since walking is usually measured in the forward direction, the distinction between speed and velocity is not significant. On level ground people without pathology consistently walk at a preferred/comfortable or “self-selected” walking speed. This speed is the most efficient for that person; faster or slower speed will cost more energy.49,50 In the gait laboratory, this preferred speed is referred to as “free walking velocity” to be distinguished from “fast walking velocity.”
Walking speed as a functional measure is highly reliable and sensitive regardless of the method of measurement. In a group of frail elderly individuals, Van Iersel demonstrated that 5% change in walking speed had a sensitivity of 92% to detect clinically relevant change.51 Walking speed also correlates with functional ability and physiological changes.52 Several studies demonstrated that walking speed is a predictor of important aspects of health status and future events including hospitalization, discharge location, and mortality.53–57
Numerous factors contribute to walking speed including joint mobility, muscle strength, sensory function, neural control, cognitive status, and energy level, so it can reflect overall health. Fritz and Lusardi suggested considering walking speed as the “sixth vital sign” in older adults. The NIH Toolbox for the Assessment of Neurological and Behavioral Function includes walking speed as a measure of motor function.58
In gait laboratory, researchers have used a variety of state-of-the-art equipment including portable computerized walkways, motion analysis systems, and foot switches to measure walking speed. Clinicians, however, can reliably measure walking speed in almost any clinical setting using a stopwatch and a walkway. Most published reports measured walking speed for the middle 6 m of a 10-m walkway to avoid the acceleration and deceleration phases and capture the steady walking speed. Suggested walkway distance varied greatly between studies, however; a walkway as short as 6 m (recording zone is the central 4 m) is still a reliable measure (NIH). Fritz and Lusardi suggest a 10-m test with added 5 m for acceleration and 5 m for deceleration.59
In people without pathology, several factors affect walking, including age, gender, lower extremity length, strength, and spontaneous variability between individuals.59 To follow the International Standards of Measurement, Gait Speed should be expressed in m/sec. Collectively the range for normal walking speed for adults is between 1.2 and 1.4 m/sec. Others reported walking speeds in m/min to be compatible with other energy and cadence measurement. Waters and colleagues reported a similar average of 82 m/min for adults.60
Functional Ambulation Classification
Holden and colleagues42 suggest that grouping subjects by motor ability or functional category is more important than grouping by other indicators of gait. They have developed the Massachusetts General Hospital (MGH) FAC.61 Six functional categories are defined in their system. A score of 0 indicates nonfunctional ambulation. Patients who require significant and constant assistance of another person for support and balance receive a score of 1. A score of 2 indicates that light touch or intermittent physical assistance is required, and 3 means that the patient needs verbal cueing or occasional safety assistance. To be scored as a 4, the patient must be independent in ambulation on level surfaces, and a score of 5 means the patient is independent in ambulation on level and nonlevel surfaces, including stairs and inclines. Although the FAC is a general ambulation test, its scores showed a positive linear relationship with such variables as gait velocity and step length.
The FAC has been used most extensively in the assessment of functional locomotion and as a rehabilitation outcome measure for individuals recovering from stroke.62–64 In a Rausch analysis of discriminant validity of measures used to assess outcome of stroke rehabilitation intervention, however, FIM motor scores and walking speed were better discriminators of outcome than FAC scores.65 The FAC has also been helpful in assessing concurrent reliability of new mobility measures in stroke rehabilitation and portable instrumented pressure-sensitive monitors.66,67
Functional Independence Measure
Initially developed to assess burden of care for staff caring for patients in acute care settings, the FIM is a multidimensional scale that assesses locomotion as one dimension of overall functional status.68 In the last decade, it has come into wide use as a tool to assess outcomes of rehabilitation interventions for patients with musculoskeletal conditions such as hip fracture and total joint replacement and for those with neuromuscular conditions such as spinal cord injury, myelopathy from spinal stenosis, and stroke.69–75 The WeeFIM is a version of the FIM intended for use with children with cerebral palsy and other developmental disabilities, acquired brain injury, and other neuromuscular and musculoskeletal impairments.76–79 The reliability and validity of the FIM have also been assessed in home care and assisted living settings for frail and disabled older adults.80,81 There is both a performance-based and interview-based version of the FIM.82,83
The FIM has two domains of function: motor and cognitive.82 The motor subscale has four dimensions: self-care (eating, grooming, bathing, dressing upper body, dressing lower body, and toileting), sphincter control (bladder and bowel management), ability to transfer (bed-chair-wheelchair, toilet, tub or shower), and locomotion (ambulation or wheelchair use, stair management). The cognitive subscale includes communication (comprehension and expression) and social cognition (social interaction, problem solving, and memory). The range of possible scores for each component of the subscales is 1 (total assistance required) to 7 (completely independent without any assistive device). Each component has specifically defined criteria. The scoring criteria for the ambulation component of the locomotion subscale are summarized in Table 5-1. The evidence of the measure’s validity is strongest when a composite FIM score or motor/cognitive subscale scores are used, although component scores are sometimes used in documentation and record keeping.81
Table 5-1
Scoring Criteria for the Locomotion Component of the Functional Independence Measure
Score | Score Criteria |
7 | Able to walk at least 150 feet (50 m) completely independently, without any type of assistive device or wheelchair, safely and within a reasonable (functional) period of time |
6 | Able to walk at least 150 feet (50 m) independently, but requires an assistive device (orthosis, prosthesis, wheelchair, special shoes, cane, crutches, walker), or takes more than reasonable time, or has safety concerns |
5 | Requires standby supervision, cueing, or coaxing to walk or propel wheelchair at least 150 feet (50 m) |
4 | Requires minimum contact assistance (patient contributes 75% effort) to walk or propel wheelchair at least 150 feet (50 m) |
3 | Requires moderate assistance (patient contributes 50% to 74% effort) to walk or propel wheelchair a minimum of 150 feet (50 m) |
2 | Requires maximal assistance of one person (patient contributes 25% to 49% effort) to walk or propel wheelchair 50 feet (17 m) |
1 | Requires total assistance (patient contributes less than 25% effort) or requires assistance of more than one helper, or is unable to walk or propel wheelchair at least 50 feet (17 m) |
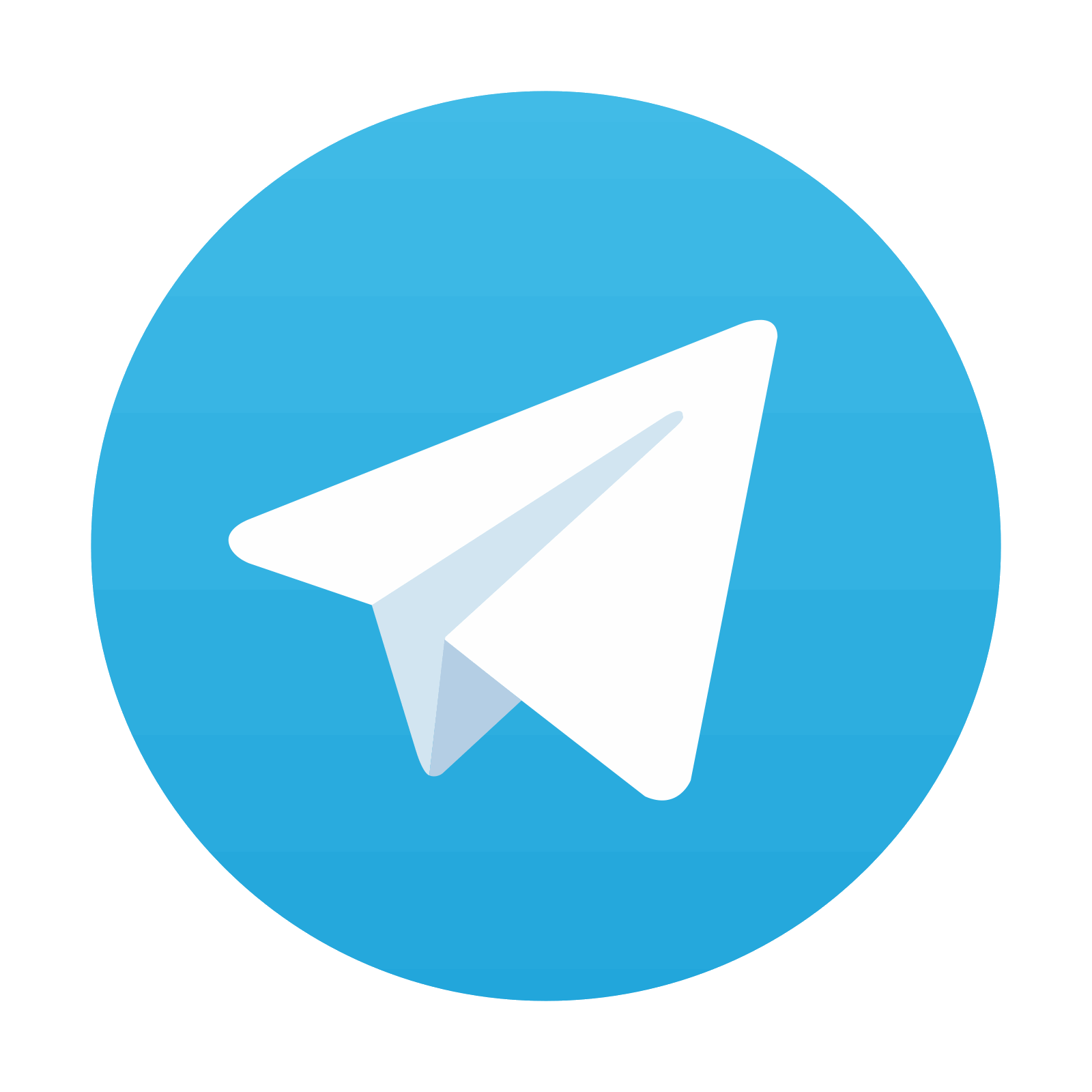
Stay updated, free articles. Join our Telegram channel
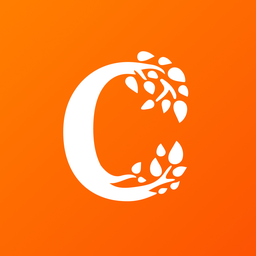
Full access? Get Clinical Tree
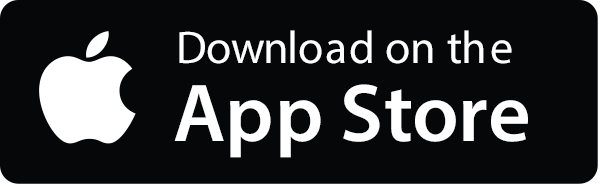
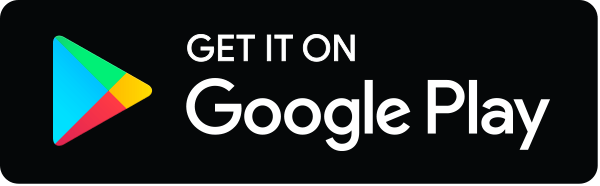