Chapter 2 Osteoporosis is characterized not only by a reduction in bone mass but also by alteration in the architecture of the bone. Changes in bone mineral density have long been considered the most important factor in the diagnosis of osteoporosis and in predicting the risk of fractures caused by osteoporosis. However, recent studies have indicated that there is an overlap on bone mineral density between the groups with and without fracture due to osteoporosis and that bone density measurement alone is insufficient to evaluate the mechanical properties of osteoporotic bone. Changes in the trabecular architecture of the osteoporotic bone are well known, but quantitative analysis of trabecular structure has not been well studied until recently. Recent rapid progress in the noninvasive measurement of the bone density and structure, such as dual photon energy X-ray absorptiometry, quantitative computed tomography, and quantitative ultrasound measurement, provides better understanding of the characteristics of osteoporosis and indicates the potential for predicting the mechanical properties of osteoporotic bone and the fracture risk. Mechanical properties of bone can be described at different levels of the bone structure, from a macroscopic level to an ultramicroscopic level, and under different mechanical basic assumptions, such as heterogeneous or homogeneous and isotropic or anisotropic assumptions.1–4 To correctly interpret the measurements from the various noninvasive techniques for osteoporosis, it is essential to understand the hierarchical structure of bone and different approaches to the biomechanical properties of osteoporotic bone. This chapter describes the hierarchical structure of bone and the mechanical properties of osteoporotic bone determined by the changes in the structure at the different structural levels. Bone exhibits hierarchical composite structure at different structural levels.1–4 The hierarchical structures are illustrated in Figure 2–1, which shows a contact radiograph of the longitudinal section of the distal femur. At the macroscopic level, the distal femur includes the diaphysis consisting of cortical bone, metaphysis where the cortex transforms to cancellous bone, and epiphysis consisting mainly of cancellous bone and shell-like cortical bone. In the epiphyseal region, higher-density cancellous bone areas are observed in the region between the cortex in the metaphysis and the loading area of the joint surface. The cancellous bone in these regions is highly oriented in the longitudinal axis of the femur. Density distribution is heterogeneous when the entire epiphysis is evaluated. However, trabecular structure can be assumed to be homogeneous within a small region of interest (ROI). Even though trabecular structure can be treated as homogeneous in a small region, clear directionality is still observed in the region. The approach considering the directionality, but not considering the microstructure of individual trabeculae, is called the homogeneous-anisotropic approach. If the directionality is not considered, this approach is called the homogeneous-isotropic approach. Usually, material properties of metals are analyzed under the homogeneous and isotropic assumption. In the heterogeneous approach at the microstructural level of cancellous bone, the individual trabecular structure, such as thickness and connectivity of individual trabeculae, is of interest. At the ultramicrostructural level, bone tissue can be described as a composite material consisting of collagen fiber and hydroxyapatite. FIGURE 2–1 Soft X-ray image of a coronal section of the distal femur showing dense cancellous bone originating from the metaphysis and oriented toward the joint surface. The following sections describe the mechanical properties of the cortical bone and cancellous bone at each hierarchical structural level and their changes in mechanical properties associated with osteoporosis. Age-related changes in macroscopic geometry of the diaphysis have been well studied. Bone resorption occurs with age on the endosteal surface of the diaphysis of the long bone. On the periosteal surface, the diameter of the diaphysis is increased with age by deposition of new bone. These age-related changes cause a change in a cross-sectional geometry of the diaphysis. When the increases in outer diameter and inner diameter are similar, the cross-sectional area remains almost constant but polar moment of inertia and area moment of inertia increase based on the increases of outer and inner diameters. When only the inner diameter of the diaphysis increases with age, the cross-sectional area decreases and moment of inertia also decreases. Although there are controversies on differences between gender in the changes in outer diameter with age,5–8 Ruff and Hayes9 demonstrated greater expansion of the outer diameter in men and noted that this age-related increase in bone cross-sectional geometry appears to “compensate” for age reductions in material properties of bone tissue at the microscopic level in men. Regardless of the differences in gender, the changes in outer diameter and inner diameter with age cause changes in cross-sectional geometry, which in turn affect bending and torsional characteristics of the whole bone. The direction of the primary osteons is generally parallel to the long bone axis. In the transverse plane, there is no specific directionality in terms of the arrangement of the osteon. It has been demonstrated that cortical bone is stronger and stiffer in the osteonal direction than in the transverse direction.3,4 The stress-strain behavior of cortical bone is dependent on the osteonal direction with respect to the loading direction. This type of material is called an anisotropic material. A composite model with isotropic assumption in the transverse plane and anisotropic assumption in the long axis of the bone based on the osteonal direction has been used to investigate the anisotropy of elastic modulus and strength of the cortical bone. The osteoporotic change in the microstructure of the cortex is seen as an increase of the porosity in the microstructural level, which reflects a decrease of the bone mineral density. Linear relationships between the bone mineral density versus elastic modulus and bone mineral density versus ultimate strength were reported.4 Decreases with age in mechanical properties of cortical bone were reported by Burstein et al.10 Yield stress, ultimate stress, and elastic modulus in tension decrease 2.2, 2.1, and 1.5%, respectively, per decade over the age range 20 to 90 on femur.10 The anisotropic properties of elastic modulus and strength are not remarkably influenced by osteoporotic changes in microstructure of the cortex.4 In the ultramicroscopic approach, the structures of the osteon and interstitial lamellae, orientation of the collagen fiber, and hydroxyapatite crystals are of interest. The strength of the interstitial lamellae is reported to be higher than that of secondary osteons.11 The number of secondary osteons increases with age.12 While osteons with longitudinally oriented collagen fibers are stronger in tension than osteons with transversely oriented fibers,13 the osteons with transversely oriented collagen fibers are stronger in compression than the osteons with longitudinally oriented fibers.14 Changes with osteoporosis in the collagen fiber orientation in the laminar structure of the osteon are still unknown. Fracture can be classified by characterizing the factor that caused the fracture (Tables 2–1 and 2–2). Fractures caused by direct forces (Table 2–1) can be sub-classified according to the magnitude and area distribution of the force, as well as according to the rate at which the force acts on the bone. Soft tissue injury and fracture comminution are related to the loading rate. Trauma energy is dependent upon the second power of loading rate. Trauma energy will be released when a bone fractures. Fractures due to indirect forces are produced by force acting at a distance from the fracture site. When a long bone is loaded, each section of the bone will be subject to both normal and shear stress. When these stresses exceed the limit of the bone, the bone will fracture. Different loads will generate different normal and shear stresses along different orientation planes within the bone. Judging the morphology of fracture lines, it is possible to predict the type of indirect injury mechanism (Fig. 2–2; Table 2–2). In general, depending upon the material strength influenced by the osteoporotic changes, the three principal stress planes (maximum tensile stress plane, maximum compressive stress plane, and maximum shear-stress plane) dictate the fracture plane and predict when and how the material will fail. Cortical bone as a material is generally weak in tension and shear, particularly along the longitudinal plane, due to the anisotropic characteristics of the strength. However, bone density change as a result of osteoporosis can also greatly reduce its material strength although the structural strength may be less apparent due to cortex geometry alteration. The failure patterns of long bones follow basic rules. Under bending, the convex side is under tension and the concave side under compression. Because bone is more susceptible to failure in tension than in compression, the tension (convex) side fails first. Tension failure occurs progressively across the bone, creating a transverse fracture without comminution. Occasionally, the cortex under compression breaks due to shear stress before the tension failure progresses all the way across the bone: the resulting comminution on the compression side often creates a single “butterfly” fragment or multiple fragments. Under torsional injury, there is always a bending moment involved that prevents the propagation of endless spinal fracture line. Shear stress may cause small longitudinal cracks on the spinal fracture line. Under experimental conditions, an average fracture angle of spinal fracture is approximately 30 degrees of the longitudinal axis, and combined axial loading has little effect on the torsional properties of whole bone.15
BIOMECHANICS OF OSTEOPOROTIC
BONE AND FRACTURES
HIERARCHICAL APPROACH TO MECHANICAL PROPERTIES OF BONES
MECHANICAL PROPERTIES OF CORTICAL BONE
MACROSCOPIC APPROACH TO MECHANICAL PROPERTIES OF CORTICAL BONE
MICROSCOPIC APPROACH TO MECHANICAL PROPERTIES OF CORTICAL BONE
ULTRAMICROSCOPIC APPROACH TO MECHANICAL PROPERTIES OF CORTICAL BONE
FRACTURE MECHANICS OF CORTICAL BONE
Type of Force | Description of the Force | Fracture Characteristics |
---|---|---|
Tapping force | Small force on a small area | Nightstick fracture of ulna |
Crushing force | High force acting on a large area | Crush fracture and severe soft tissue injury |
Penetrating force | High force acting on a small area | “Open” fracture and minimal to moderate soft tissue disruption |
Penetrating-explosive force | High force acting on a small area at a high or extremely high loading rate | “Open” fracture and minimal to moderate soft tissue disruption and devitalized bone fragment |
Fracture Type* | Example of Fractures | Injury Force |
---|---|---|
Transverse | Some transverse patella fractures | Tensile force |
Oblique | Oblique or Y-fracture of the distal femur/humerus | Axial compressive force |
Spiral | Spiral fracture of the the tibia/humerus with intermittent longitudinal crack lines | Torsional force |
Transverse | Transverse shaft fracture of the humerus/tibia with small butterfly fragment | Bending force |
Transverse oblique | Transverse shaft fractures of the tibia with large butterfly fragment | Axial compression and bending |
*Fracture types are graphically demonstrated in Figure 2–2 from right to left.
FIGURE 2–2 Typical long-bone fracture morphology corresponding to the type of external load applied to the long bone. The fracture pattern may vary depending upon the magnitude of the composite loading mode involved.
The susceptibility of bone to fracture with a single injury is related to its energy-absorbing capacity and modulus of elasticity. The loading rate of bone affects its energy-absorption capacity. Bone undergoing rapid loading will absorb more energy than when loaded at a slow rate.16 However, at very high loading rates there is a decline in energy absorbed.15 The energy absorbed by bone during loading is released when the bone fractures. This phenomenon helps to explain why injuries with rapid loading involving higher velocities dissipate greater energy and result in greater fracture comminution and displacement.
Clinically, it is well known that spinal and oblique fractures tend to heal faster than some transverse fractures. This difference in the inherent healing rate has been commonly related to as the difference in the amount of soft tissue destruction; that is, in the difference of injury mechanism and fracture energy.17 Another variable is the increased surface area of fracture ends in oblique/spinal fractures. In vitro experiments have considered the difference in fracture energy under transverse and spinal failure of a loaded bone. Analysis of the load-displacement curves showed no statistically significant difference in the amount of energy absorption at failure (Fig. 2–3). However, this difference may change as the base material properties and geometry alter as a result of aging and osteoporosis. Such change is due to the different stiffness properties of the bone under torsion and bending. Regardless of bone material and geometry changes, the larger load under bending failure may cause the surrounding soft tissues and periosteum to sustain more damage and thus affect bone fracture healing potential. This information is useful in fracture fixation device design and in the care of patients whose bones have undergone significant osteoporotic changes.
The susceptibility of bone to fracture under fluctuating forces (or stresses) is related to its crystal structure and collagen orientation, which reflects the viscoelastic properties of the bone. Cortical bone is vulnerable to both tensile and compressive fluctuating stresses. Under each cycle of loading, a small amount of strain energy may be lost through microcracks along the cement lines. Fatigue load under certain strain rates can cause progressive accumulation of microdamage in cortical bone. When such a process is prolonged, bone may eventually fail through fracture crack propagation. It is expected that osteoporotic bone will have poor fatigue resistance. However, it is still a living tissue and can undertake a repair process although such reparative process may be inferior when compared to that in normal bone. Periosteal callus and new bone formation near the microcracks can arrest crack propagation by reducing the high stresses at the tip of the crack. However, for this repair process to be effective, a relatively low level of stress must be applied and maintained on the bone.
FIGURE 2–3
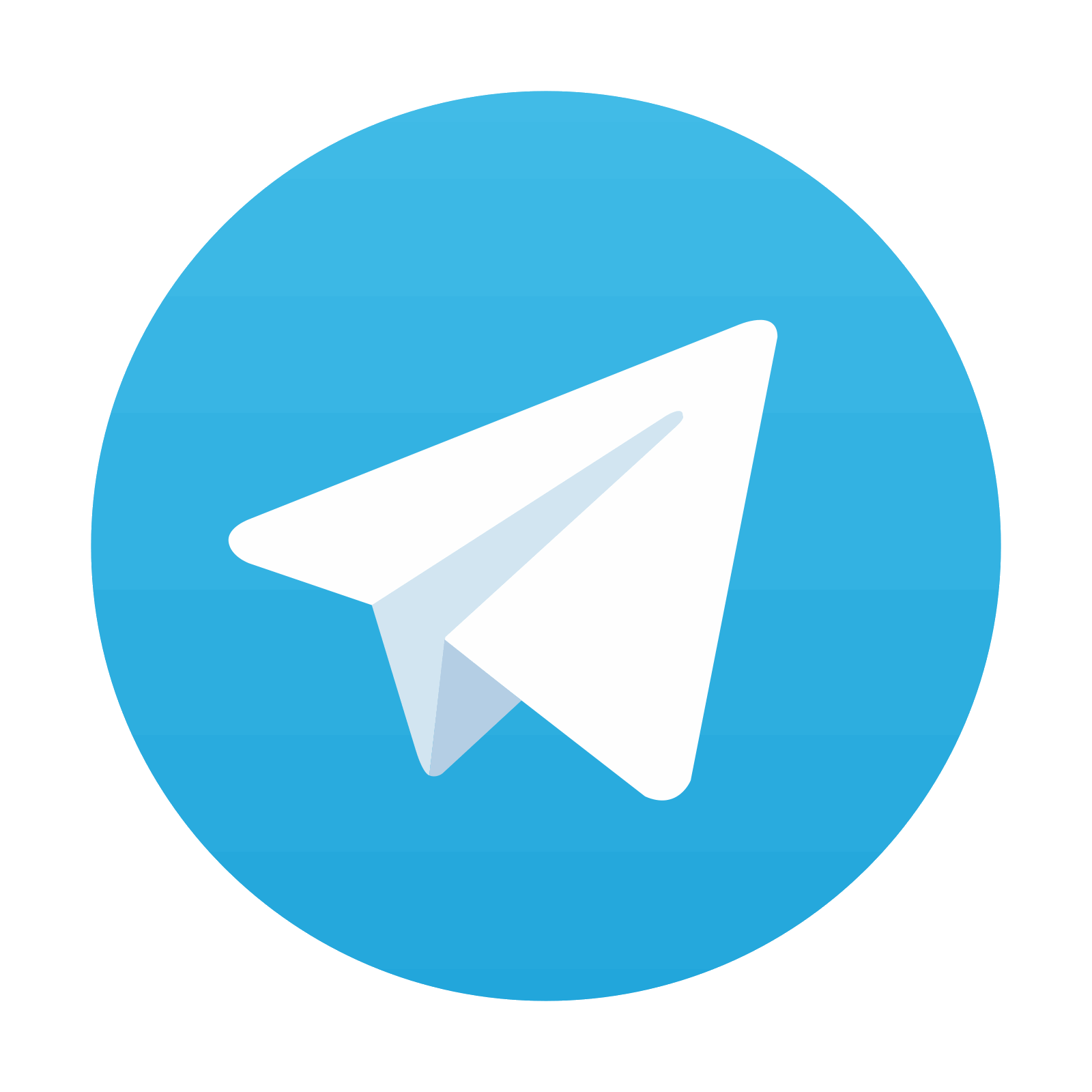
Stay updated, free articles. Join our Telegram channel
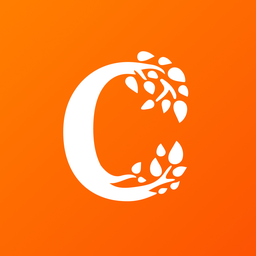
Full access? Get Clinical Tree
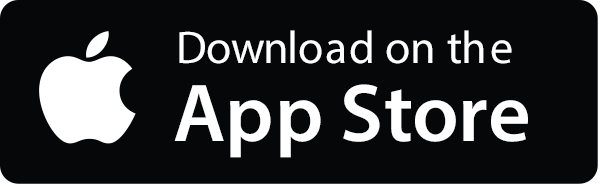
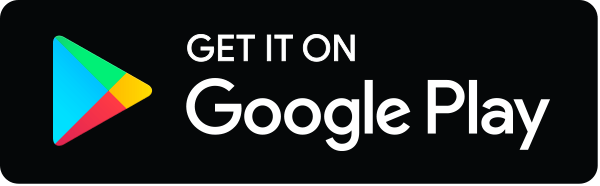