Chapter 3 Osteoporosis is a systemic disease characterized by a decreased bone mass and reduction in mechanical properties. Osteoporotic fractures and related health problems and costs represent a significant and growing problem. An increase in age-adjusted incidence of all fragility fractures, hip, vertebral, and radius, has been seen in the Western world.1 The projected costs of osteoporotic fractures in white post-menopausal women during the next 10 years in the United States alone is estimated at more than $45 billion.2 More than 5.6 million fractures have been estimated to occur each year in the United States alone3 and somewhere between 5 and 10% of these result in delayed or impaired healing.4 Fracture healing is a complex biological cascade characterized by the sequential expression of fibrous, cartilaginous, and bone-specific genes. Our understanding of the molecular events during fracture healing have benefited greatly with recent advances in molecular biology techniques. A thorough understanding of the cellular and molecular events and the correlating mechanical properties remains unknown. The events in fracture healing in the aged and osteoporotic patient are confounded by a known decrease in bone formation in osteoporotic patients compared to age-matched controls.5,6 The effect of estrogen deficiency on fracture healing remains controversial. The ability to augment or accelerate fracture healing has the potential to play an important role in the clinical treatment and economic considerations in fracture management. This chapter will examine fracture healing and the biological and mechanical factors that have been suggested to play an important role and to have the potential to provide a clinical treatment. The effects of estrogen deficiency on histology and immunohistochemistry fracture healing will also be examined. Fracture healing has long fascinated and amazed researchers and clinicians. The unique concert of events that follow the initial injury play a vital role in the dynamic ability of bone to regenerate and repair itself and have been the source of a multitude of studies. Recent data suggest the regenerative capacity of adult bone may depend upon the reinduction of the pathways from fetal development of chondrogenesis and osteogenesis.7–9 Fracture regeneration aims to provide stability to the injury site to allow the infiltration of a new vascular system and ultimate remodeling to a mature and load-bearing construct. The pathways for bone regeneration are related to the mechanical boundary conditions, stability and imposed loads, at the fracture site. Motion at the fracture site will lead to a cartilaginous pathway via endochondral ossification indirect, secondary healing, whereas a stable mechanical environment leads to bone formation without a cartilaginous intermediate direct, primary healing. Primary bone healing follows a similar sequence of events observed for filling of cortical bone defects.10 The initial phase provides a scaffold of woven bone that is later remodeled to Haversian or secondary bone. This type of healing has been observed following the principles of open reduction and internal fixation (ORIF). Studies describing the histological features of secondary fracture healing11–18 have evolved from the early analogy of blastema production.19 Healing or regeneration of a low-energy long bone fracture via secondary healing has been described in five phases: immediate injury, intramembranous bone formation, chondrogenesis, endochondral ossification, and bone remodeling.20–23 Immediately following the mechanical insult and formation of hematoma there are two separate, but simultaneous events: (1) intramembranous ossification via proliferation of osteoprogenitor cells from the cambial layer of periosteum, and (2) endochondral ossification from the chondrogenesis of undifferentiated mesenchymal cells in the soft tissues adjacent to the fracture site and in the bone marrow. In simple terms, a fracture results in fragments of dead bone and disruption of soft tissues and local vascular and nutrient supply. Activated platelets release a variety of products to the local environment that have been thought to play a role in fracture healing and bone repair, including platelet-derived growth factor (PDGF), transforming growth factor beta (TGF-β), and osteocalcin.24 The ensuing influx of inflammatory cells that secrete fibroblast growth factor and additional PDGF and TGF-β and cytokine cascade interleukin (IL)-1 and IL-6 brings the cells of repair, fibroblasts, osteoblasts, and endothelial cells, into the fracture site, which may be important in regulating the early events in fracture healing.25 Some of the earliest changes as a result of the milieu of factors were reported by Brighton and Hunt in a rabbit model.26 These authors noted the early loss of normal architecture of the marrow and the disappearance of blood vessels in the region of high cellular density adjacent to the fibrin clot in the medullary callus; the enlargement and transformation of capillary and venous endothelial cells in the region of low cellular density adjacent to the normal, uninjured marrow; the appearance of polymorphic mesenchymal cells throughout the medullary callus; and the appearance of osteoblasts and new bone formation by 24 hours after the fracture.26 New bone matrix is synthesized by osteoblasts adjacent to the fracture site and below the proliferating periosteal cells. This primary bone forms without a cartilaginous intermediate via an intramembranous pathway. This phase is characterized by chondrogenesis by mesenchymal cells and an enlarged cartilaginous matrix at the fracture site soft callus that provides some initial stabilization. The presence of fibrous tissue as well in the fracture site adjacent to the cartilaginous matrix is commonly seen. The move into the formation of hard callus and union at the fracture site is achieved as a result in woven bone formation. This weak woven bone forms through a cartilaginous intermediate as the chondrocytes undergo hypertrophy7,27 and produce and release neutral proteases that degrade the cartilage matrix, including proteoglycans prior to mineralization.28,29 The final phase of healing represents the final regeneration from disorganized woven bone to a return to Haversian bone prior to the initial event. Improvements in clinical treatment of fractures may come as research into the molecular mechanisms behind the fracture cascade of events are understood. Many local and systemic regulators are involved in fracture healing, including growth and differentiation factors, cytokines, hormones, and extracellular matrix components.30–35 The expression of these factors appears to be spatially and temporally restricted, suggesting a fine level of control and balance during fracture regeneration and healing. The following summarizes a number of findings related to the proteins expressed during fracture healing. Collectively, these studies reveal the fine level of control of the biological mechanisms. A number of new and unique molecules have recently been identified that will no doubt bring about new questions and research. A number of excellent review articles are available for an overview of growth factors as they relate to fracture healing.4,25,30,36,37 The temporal expression of a number of extracellular matrix (ECM) proteins during fracture healing have been reported in the hard and soft callus,38,39 as well as the spatial expression of many of these molecules through in situ hybridization. These proteins include a variety of collagen types (Col I, II, V) as well as a number of noncollagenous proteins, including osteonectin (ON), osteocalcin, osteopontin, and alkaline phosphatase (ALP). Using Northern analysis to determine the relative level of gene expression for intracellular matrix proteins gene, Jingushi and Bolander38 demonstrated that a temporal expression of different ECM protein genes can be correlated with cellular events and the phases of fracture healing, including the initiation of intramembranous bone formation, endochondral ossification, as well as bone remodeling. Changes in the ECM protein gene expression in the hard and soft callus differed further, demonstrating the differences between these tissues at the local molecular level. The sequential expression of growth factors during fracture healing confirms the dynamic nature of the fracture cascade. A number of growth factors have been detected in the healing fracture including fibroblast growth factor (FGF), PDGF, insulin-like growth factor (IGF), and TGF-β.20,40 PDGF and TGF-β are among the first factors to be present following their release from degranulating platelets, followed by basic FGF and additional PDGF and TGF-β. A number of authors have reported using immunohistochemistry to detect the presence of growth factors as well as bone morphogenetic proteins (BMPs) during the fracture cascade in the standard closed fresh fracture model.20,38,40,41 Little data to date has been presented on a similar comparison in the estrogen-deficient fracture model. Members of the FGF family have been reported to have a diverse range of functions that have been reviewed elsewhere.42,43 Both the acidic and the basic forms of FGF have been detected during the early stages of fracture repair38,40 and are implicated in the regulation of cartilage and bone. PDGF, synthesized by a number of cell types, including platelets, macrophages, monocytes, and endothelial cells, has also been shown to be a potent mitogen for all cells of mesenchymal origin. PDGF has been detected during fracture healing using immunohistochemistry as well as in situ hybridization. Andrew et al44 have reported PDGF expression by a number of cell types for prolonged periods during fracture healing. Among these factors, the TGF-β/activin/BMP cytokine family has been implicated to play an important role in bone formation and healing. BMPs have been detected during ectopic bone formation in vivo, and they influence mesenchymal cell proliferation, differentiation, chondrogenesis, and osteogenesis in vitro,45–47 whereas TGF-β is involved in wound healing and tissue repair.48 Both factors initiate their biological effects from the cell surface through two related serine-threonine kinase receptors, the type I and II receptors.49 Upon ligand binding, the activated type II receptor phosphorylates/activates the type I receptor. In turn, the activated type I receptor propagates the signal to downstream substrates.50 TGF-β’s role in fracture healing begins with the initial response and release from degranulating platelets. Joyce et al,21 using immunohistochemical and recombinant DNA techniques, have implicated TGF-β as an important regulator of cell proliferation, differentiation, and synthesis of extracellular matrix proteins during fracture healing. TGF-β has been localized in regions of cell differentiation and proliferation and is synthesized by these cells. TGF-β1 gene expression was noted to peak at day 13 in the soft callus, whereas the hard callus presented a maximal response at day 5 and a submaximal peak at day 15. Rosier and coworkers reported the temporal expression of different TGF-β isoforms in a chick fracture model.41 The pattern of TGF-β1 expression has been reported by many investigators.21,51 Brager et al52 provided the local mRNA expression of a number of growth factors in the callus tissue at 7, 14, 21, and 28 days using the rodent fracture model.53 These authors did not differentiate between hard and soft callus. Expression levels were relative to aβ-actin control revealed peak expression of TGF-β 1–3 at day 14, which remained relatively high at day 21 and decreased by day 28. FGF-1, FGF-2, and FGF-9 were noted to peak on days 14, 21, and 28, respectively, whereas BMP-2 expression increased from day 7 to 14 and remained relatively constant. Andrew et al44 investigated IGF-I and III mRNA expression in normally healing human fractures by in situ hybridization. Endothelial and mesenchymal cells at the early phases of healing granulation expressed IGF-II mRNA. Expression of IGF-I mRNA was detected during endochondral and intramembranous ossification. These results support a role for IGFs in local cellular regulation during fracture repair. The presence of matrix metalloproteinase-13 (MMP-13) during fracture healing has recently been reported using a mouse rib fracture model.54 MMP-13 (collagenase-3) is a member of the MMP family, which degrades type II collagen about sixfold more effectively than type I or type III collagen. MMP-13 mRNA was present in the cells of the periosteum at day 1 as well as in the cells of the cartilaginous phase and hypertrophic chondrocytes and immature osteoblastic cells in the fracture callus. Murakami and Noda55 reported the expression of Indian hedgehog (Ihh) in chondrocytes and osteoblasts during fracture healing using a standard rodent fracture model53 at 1, 2, and 3 weeks following fracture. Ihh expression was known to be enhanced by TFG-β, which provided motivation to examine its role in fracture healing.55 These findings indicated that this developmental factor is expressed during fracture healing and could participate in the regulation of endochondral bone formation at the fracture site. Asaumi et al56 reported the expression of neurotrophins and their receptors in a mouse rib fracture model. Expression levels of three neurotrophins were increased during fracture healing. Peak levels were found as early as 2 days after fracture. Neurotrophins and their receptors exist in bone-forming cells, which suggests they may play an important role in the regulation of bone formation as autocrine or paracrine factors in vivo.56 Since the key discovery by Urist,57 BMPs have been the source of great interest in fracture healing. BMPs are pleiotropic regulators of chemotaxis, mitosis, and differentiation.58,59 To date, at least 16 BMPs have been identified.60,61 Several studies have reported62–64 the presence of BMPs during fracture healing as early as day 3 using a rabbit mandible fracture model. Bostrom and coworkers65 have confirmed the presence, localization, and temporal expression of BMP-2 and BMP-4 using a standard closed adult rodent fracture model53 at 2, 4, 6, 8, 10, 14, and 21 days following fracture. BMP-2 and BMP-4 expression appeared to be confined to primitive osteoblastic and chondroblastic tissues.65 Ishidou and coworkers66 have also reported the expression of BMP-2, BMP-4, and BMP-7 and osteogenic protein 1 (OP-1) during fracture healing. BMP-7 demonstrated a different localization and temporal expression pattern compared to BMP-2 and BMP-4. Individual BMPs may have different functions during the healing process, which has yet to be completely understood. The expression of BMP receptors during fracture healing has also been reported using the adult closed fracture model.53,66 BMP type IA and IB receptors are expressed in osteoblasts and periosteum and are up-regulated in the osteogenic cells of the periosteum near the fracture ends. These receptors also demonstrated a temporal and specific expression pattern, again suggesting different roles for these receptors during fracture healing. Activins are multifunctional proteins that belong to the TGF-β superfamily and are thought to play an important role in modulating the formation of bone.67 Activins stimulate erythroid differentiation of hematopoietic progenitor cells of bone marrow68 and are involved in embryonic development.69 Activins are dynamically regulated by follistatins, which are monomeric glycoproteins. Activin A and follistatin have been detected using immunohistochemical techniques in ectopic bone formation in subcutaneous demineralized bone matrices.67 Activin A and follistatin are coexpressed in prechondrocytes, with the expression decreasing with maturity. Furthermore, the process of endochondral ossification has been shown to be inhibited by injections of rh-follistatin. The activin-follistatin system therefore may play an important role in bone development. Nagamine and coworkers70 recently reported the immunohistochemical expression of activin A, follistatin, and activin receptors during fracture healing using the standard rat model.53 Activin and follistatin were not expressed in normal intact femurs of the rat. Activin and follistatin, however, were detected at the fracture site. Immunostaining for activin A was initially weak in the osteogenic cells and extracellular matrix of the thickened periosteum adjacent to the fracture site, as well as the fibroblastic-like cells around the hemotoma on day 3. Staining for follistatin was strong in the periosteum. By day 7 after fracture, activin A expression decreased below levels of detection, whereas follistatin was weakly expressed. Activin staining also remained weak in the fibroblast-like cells located in the periphery of the cartilage islands on days 7 and 14. Follistatin was expressed moderately or strongly in the proliferating, mature, and hypertrophied chondrocytes on days 7, 14, and 28. Activin A staining was also noted in the osteoblasts and bone matrix of newly formed trabecular bone. Sakai et al71 reported that the local administration of activin promotes fracture healing in a rat fibula model. The mechanism of action of BMPs has evolved following the identification of a novel family of proteins, the Smads, as TGF-β/activins/BMP signal transducers.72–74 Smads are the vertebrate homologs of Mad Mothers against decapentaplegic gene from Drosophila and/or Sma genes from Caenorhabditis elegans. They are categorized into three classes based on their different functions. Smad1, Smad2, Smad3, Smad5, and Smad8 belong to the receptor-regulated class (R-Smad), which is activated by the type I receptors.72–74 Smad2 and Smad3 are initiated by TGFß/activin and induce the responses to their ligands, while Smad1, Smad5, and Smad8 mediate BMP responses. This class of Smads forms heteromers with the common-mediator class (Co-Smad): Smad4, moving from cell surface to nucleus, positively regulates target gene function. Smad6 and Smad7 (inhibitory Smads or anti-Smads) perform negative regulatory or balancing roles in those processes.72–74 Smad1, Smad2, and Smad5 have been found to be highly expressed in proliferating chondrocytes, while Smad3, Smad4, Smad6, and Smad7 were strongly exhibited in mature chondrocytes in epiphysis of growing rats.46,75 In vitro studies by Fujii et al76 have shown Smad1 and Smad5 can induce alkaline phosphatase activity in C2/C12 mouse myoblast cells and chondrogenic differentiation of ATDC5 cells, whereas Smad6 and Smad7 repressed this activity. These studies suggest that Smads play a role in osteoblast and chondroblast differentiation and proliferation as well as endochondral ossification.59,60 Smad expression during fracture healing in normal or diseased states has not been reported. In a pilot study, we examined the expression of Smad1, Smad4, and Smad5 during fracture healing in normal and estrogen-deficient state (see below). Accelerating or enhancing fracture regeneration has the potential to influence the clinical decisions in fracture management as well as the economic burden on society. The aim of enhancement may be to accelerate a fracture that is likely to heal, or to assure the healing of a fracture that is not likely to heal without extraordinary measures.22 Either scenario has the potential to reduce clinical and socioeconomic problems associated with fracture healing. A number of noninvasive and invasive strategies that have been developed over the years will now be considered. Noninvasive methods in fracture treatment through systemic injections or biophysical stimulation electromagnetic ultrasound have been examined. Systemic injections of L-dopa have been reported to enhance early fracture healing as well as nonunions.77,78 L-dopa is converted in the body to dopamine, which stimulates the secretion of growth hormone, which in turn can influence healing. Holzer et al79 reported the effects of daily subcutaneous injections of parathyroid hormone in a rat closed fracture model.53 Treatment with parathyroid hormone resulted in a statistically significant increase in callus area and strength. Histology also showed an increase in the amount of new bone. Biophysical stimulation, either electric, electromagnetic, or ultrasound, represents the most common clinically employed noninvasive method used to treat fractures. The development of electric and electromagnetic techniques has been based, in part, on the discovery of the piezoelectric properties of bone tissue. The foundation of these noninvasive strategies relates to strain-generated electric potentials acting as a signal for the regulation of cellular processes involved in bone repair and remodeling.80 A number of studies have examined the in vitro effects of electromagnetic stimulation.81,82 These authors have proposed that magnetic fields stimulate the secretion of IGF-II. Nagai and coworkers83 reported stimulation of TGF-β, BMP-2, and BMP-4/mRNA, respectively, following pulsed electromagnetic stimulation. Clinically beneficial effects of electrical stimulation have been shown over the past decade using a variety of stimulation techniques on nonunions and have been reviewed by Ryaby.84 Low-intensity ultrasound is another biophysical method that represents the sole intervention approved by the Food and Drug Administration to augment the healing of fresh fractures.85 A review of ultrasound-induced effects on fracture healing was reported by Hadjiargyrou et al.85 The general consensus from these studies supports the clinical use of ultrasound in fracture regeneration. Warden et al86 recently reported a review of the Sonic Accelerated Fracture Healing System (SAFHS, Exogen Inc., Piscataway, NJ). Despite the number of successful reports following ultrasound treatment in animal work, the physical process responsible for these effects still remains relatively unknown. This reflects the complexity of the fracture-healing cascade as well as limited understanding of how ultrasound signals are transduced in vivo to elicit a cellular response. Kristiansen et al87 in a multicenter, prospective, randomized, double-blind, placebo-controlled clinical study recently reported that a specific ultrasound signal accelerated the healing of fractures of the distal radial metaphysis and decreased the loss of reduction during fracture healing. Heckman et al88 have reported acceleration of tibial fracture healing in humans following low-intensity pulsed ultrasound (Exogen, Smith and Nephew Endoscopy, Andover, MA).88 A significant decrease in time to clinical healing was observed (86 versus 114 days), which demonstrates the efficacy of low-intensity ultra-sound stimulation in the acceleration of the normal fracture repair process. The economics in reduction in healing time of tibial fractures treated with ultra-sound stimulation was examined by Heckman and Sarasohn-Kahn.89 Reduced healing time yielded a substantial cost-saving in fracture treatment.89 The mechanical environment is generally accepted as having a major influence on the course of the healing fracture. Mechanical stimulation can induce fracture healing or alter its biologic pathway. Mechanical stimulation through specific, controlled degrees of induced micromotion has been shown in preclinical and clinical trials to enhance fracture healing90,91 as well as hypertrophy of bones or other connective tissues. The healing of osteotomies created in animal models have been shown to be influenced by the strain magnitude, strain rate, and stress applied to the tissues.4,90 Invasive fracture treatment strategies include the use of autogenous bone grafting and has recently moved into the field of osteobiologics. The osteobiologic approach has been hampered, traditionally, due to a lack of detailed knowledge concerning the temporal and spatial distribution of growth factors and exogenous osteoinductive agents during fracture healing and a number of issues related to delivery and release kinetics. The use of autologous bone graft remains the gold standard but has a number of well-recognized complications in terms of donor site morbidity, mechanical properties, blood loss, increased operating time, and limited supply. The choice of autogenous bone grafts or allograft bone represents a complicated clinical decision that needs to consider local biology and mechanical stability.92 Other techniques such as the use of marrow osteoprogenitor cells aspirated from the iliac crest and injected into the fracture offer other autogenous methods of invasive therapy. Another recent autologous strategy based on the isolation and concentration of platelets from the patient’s own blood has been applied to a number of bony sites. A few hundred milliliters of blood is taken prior to the procedure and the platelets and fibrinogen concentrated in a two-stage procedure known as Autologous Growth Factor (AGF) therapy (Interpore-Cross International, Irvine, CA). AGF has been examined in an ovine posterolateral spinal fusion model in combination with a resorbable ceramic and aspirated bone marrow.93 The addition of AGF to autograft increased the stiffness by nearly 15% compared to autograft alone, while the addition of AGF and aspirated marrow increased the stiffness nearly 50% compared to AGF + ProOsteon 500R (platelet concentrate and bone graft substitute; Interpore-Cross International, Irvine, CA) (P <0.05).93 The increase in osteobiologic strategies is not surprising considering the wealth of new knowledge concerning the fracture regeneration process at the molecular level. Lind37 recently reviewed a number of in vivo studies using invasive therapy through the addition of exogenous growth factors or BMPs (including PDGF, IGF, BMP-3, BMP-7, TGF-β) in a variety of animals (rat, rabbit, dog, sheep, baboons, monkeys). Early studies on the effect of a single growth factor are plagued with questions concerning dose, timing, and release kinetics. Reddi58 reports increased understanding of the molecular cascade of growth factors following a fracture. Jingushi and Bolander38 have helped provide rationale behind the factor chosen and delivery method. The work of this group on correlating the changes in the histology of the fracture callus with changes in gene expression provides sound motivation for the delivery of different factors to the fracture site.38 The effects of the application of a single growth factor or bioactive molecule have shown variable results. This in part reflects differences between the model, surgical site, method, animal, and age, as well as the endpoints used to evaluate the healing site (radiographic, histologic, and biomechanical). Bostrom et al94 reviewed many of the articles examining the effects of the exogenous application of a single growth factor on fracture healing. Many studies report some histologic or radiographic change, with overall poor results in terms of mechanical properties. Researchers95–102 have reported the effects of different dosages of recombinant human basic fibroblast growth factor (bFGF). These authors report an optimal stimulator dose of 15 ng of bFGF per implant, whereas a higher dose had a marked inhibitory effect.95,102 Local injections of acidic fibroblast growth factor (aFGF) have been reported by Jingushi et al103 to increase the cartilage proportion of the fracture callus in the rodent fracture model. Systemic administration of bFGF at 0.1 mg/kg/d to growing rats increased endosteal and endochondral bone formation while decreasing periosteal bone formation.83 Radomsky and coworkers43
BIOLOGY AND BIOMECHANICS
OF FRACTURE HEALING
FRACTURE HEALING
NORMAL FRACTURE HEALING
HISTOLOGY PHASES
Phase 1: Hematoma Formation, Inflammation, and Granulation Phase
Phase 2: Intramembranous Bone Formation
Phase 3: Chondrogenesis
Phase 4: Endochondral Ossification
Phase 5: Bone Remodeling
GROWTH FACTORS AND BONE MORPHOGENIC PROTEINS DURING FRACTURE HEALING
EXTRACELLULAR MATRIX PROTEINS
GROWTH FACTORS AND FRACTURE REPAIR
FIBROBLAST GROWTH FACTOR AND PLATELETDERIVED GROWTH FACTOR
TRANSFORMING GROWTH FACTOR-β
MATRIX METALLOPROTEINASE-13 (COLLAGENASE-3)
INDIAN HEDGEHOG
NEUROTROPHINS
BONE MORPHOGENIC PROTEINS
ACTIVINS AND FOLLISTATIN
SMADS
ENHANCING FRACTURE HEALING
NONINVASIVE METHODS
INVASIVE METHODS
AUTOLOGOUS METHODS
GROWTH FACTORS
Stay updated, free articles. Join our Telegram channel
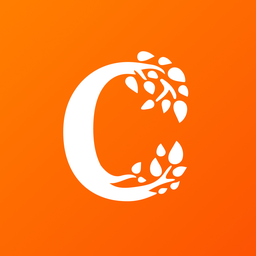
Full access? Get Clinical Tree
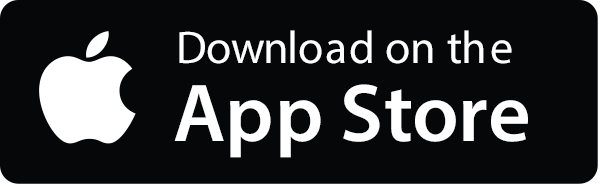
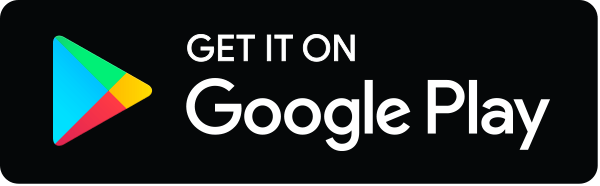