Fig. 13.1
Tendon structure (ascending order): fibrils, fibers, subfascicles, fascicles and tendon
13.2.1.1 Extracellular Matrix (ECM)
As other connective tissues, tendons are mainly composed of water (55–70% total weight) (Sharma and Maffulli 2005; Thorpe and Screen 2016). Tendon dry weight is mainly formed by ECM and cells account only for roughly 20% of total volume (Benjamin and Ralphs 2000).
The most common protein in tendons ECM is collagen, and 95% of it is collagen type I oriented mainly in a longitudinal fashion granting tendons high tensile strength (Hammoudi and Temenoff 2011; Thorpe and Screen 2016). Collagen type III, the second most common type (Thorpe and Screen 2016), is found primarily in endotenon and epitenon and, represents a less organized matrix associated with decreased strength but increased pliability. It is the main collagen type synthesized in the early phases of tendon and ligament healing (Hammoudi and Temenoff 2011; Wang 2006). Collagen type V can be found in the center of collagen type I fibrils and may contribute to fibrillogenesis.
Other types of collagen exist in lesser quantities (II, VI, IX, X, and XI), usually at insertion sites where they are thought to reduce stress over interface zones (Hammoudi and Temenoff 2011).
Elastin is another protein commonly found in tendons and ligaments reaching 2% of tendon dry weight (Sharma and Maffulli 2005). It usually bounds to collagen type I fibrils forming elastic fibers. They can store return energy and contribute to recover of fibril crimp structure after strain is applied to elastic fibers (Hammoudi and Temenoff 2011; Wang 2006).
Proteoglycans comprise 1–5% of tendon and ligament dry weight depending on location and function. Among their described functions, they maintain hydration of tendons, provide lubrication to the gliding fibers, improve adaptation and tensile strength, enhance compressive stiffness in fibrocartilaginous regions, and also have a role in tendon development (Hammoudi and Temenoff 2011; Thorpe and Screen 2016). The most common proteoglycan is decorin, making up to 80% of proteoglycan dry weight in tendons. It contributes to tendon development and is predominantly found in tensile zones, allowing tendon adaptation to tensile loads (Hammoudi and Temenoff 2011; Thorpe and Screen 2016).
Fibronectin and tenascin-C are glycoproteins found in small quantities in tendons and ligaments, and they contribute to ECM repair and mechanical stability after injury mainly by their interaction with collagen and other structural components (Hammoudi and Temenoff 2011). Moreover, Tenascin-C expression is upregulated in tendinopathy (Sharma and Maffulli 2005).
13.2.1.2 Cells
There are several types of cells present in tendons, but the majority of these cells are fibroblasts, and they are responsible for synthesizing and organizing ECM components (Benjamin and Ralphs 2000; Hammoudi and Temenoff 2011; Sharma and Maffulli 2005; Wang 2006). Fibroblasts that reside between collagen fibers, known as tenocytes, provide a complex network of cytoplasmic processes connecting adjacent cells and surrounding collagen fibers (Thorpe and Screen 2016). They are also the main cells recruited in response to injury and are able to amplify inflammatory response by secreting essential chemotactic and growth factors. Other cell types, namely, tenoblasts (tenocyte progenitor cells), chondrocytes, and synovial and vascular cells, can be found in the interfascicular space (Dyment and Galloway 2015; Sharma and Maffulli 2005).
13.2.1.3 Tendon Blood Supply
Tendon blood supply is very variable. In most of cases, tendons are supplied by intrinsic vessels (myotendinous and osteotendinous junctions) and extrinsic vessels that penetrate synovial sheath or paratenon and course deeper into the tendon through epitenon and endotenon (Sharma and Maffulli 2005).
13.2.2 Tendon–Bone interface (TBi)
TBi is a highly organized structure where two different tissues connect allowing the transmission of force produced by the muscle to the bone and resulting in movement. Briefly, it is composed of a gradual interface between tendon and bone which is composed of four zones: a dense tendon zone, non-mineralized fibrocartilage, mineralized fibrocartilage, and bone (Benjamin and Ralphs 1998).
Moreover, the tendon is composed of a highly organized collagenous extracellular matrix and parallel collagen fibers that are oriented along the long axis of the tendon. Some specialized fibroblasts (tenocytes) are distributed in parallel rows between the collagen fibers, and a few vascular structures are evident. Then, the dense collagen fibers of the tendon gradually intermesh with fibrocartilage, and the latter becomes progressively mineralized and continues into the adjacent cortical bone. There is also a distinct transition line between the mineralized and the non-mineralized fibrocartilage known as tidemark. The tidemark is a relative straight line, which indicates the production of a flat surface during the mineralization process. A histological photograph is provided showing the histological characteristics of TBi (Fig. 13.2).


Fig. 13.2
TBi histology. The normal histology of the intact TBi is composed of four consecutive zones: a dense tendon zone, non-mineralized fibrocartilage, mineralized fibrocartilage, and bone. The tendon collagen fibers are typically oriented along the long axis of the tendon and the fibrocartilage zone becomes progressively mineralized toward the bone border. There is also a tidemark, a well-defined transition line between the non-mineralized and mineralized fibrocartilage, which indicates the production of a flat surface during the mineralization process. (Mason’s Trichrome, original magnification 40×)
13.3 Injury, Healing, and Repair
The tendon healing process after injury or surgical repair is usually predictable with three overlapping stages—(1) inflammation, (2) proliferation/matrix production, and (3) remodeling (Hope and Saxby 2007; Sharma and Maffulli 2005). In this process, the recruitment and activation of intrinsic tenocytes peaks in the proliferation phase where they are recruited from the paratenon, endotenon, and epitenon, migrate to the wound site, and begin proliferating (Garner et al. 1989; Yang et al. 2013a). All sources of tenocytes are important in synthesizing an extracellular matrix and in establishing an internal neovascular network (James et al. 2008).
In the tendon healing process and more specifically in the inflammatory phase, various kinds of cells, including fibroblasts/tenocytes, platelets, and MSCs, enter the wounded area, and cellular interactions play a crucial role in the healing process (Woo et al. 1999). In particular, BMSCs contribute to the process of tendon healing (Young et al. 1998). However, in the contribution of MSCs to the healing process, it is still unclear as to whether the effects are obtained by differentiation of MSCs themselves into tenocytes at the injury site (Smith and Webbon 2005) or instead they act enhancing the local biological behaviors via cell–cell contact or by the regulative effect supplying immunomodulatory and trophic factors or whether a combination of all these mechanisms occurs (Chong et al. 2009; Lange-Consiglio et al. 2013; Yagi et al. 2010).
It is currently defended that one of the possible mechanisms of the biological effects of MSCs is from paracrine factors releasing from these cells (known as “secretome”) which can control regenerative mechanisms and improve the ability to control inflammation (Lange-Consiglio et al. 2013; Sevivas et al. 2016). In fact, it has been shown that the secretome of MSCs regulates cellular functions such as proliferation, differentiation, communication, and migration (Carvalho et al. 2011; Makridakis et al. 2013; Salgado and Gimble 2013; Salgado et al. 2015; Teixeira et al. 2013, 2015). Finally, from the clinical application point of view, trophic factors produced by stem cells in the form have shown to have reparative effects in spontaneous horse tendon injuries (Lange-Consiglio et al. 2013).
However, the tendon natural healing process occurs by reactive scar formation and does not achieve to obtain a tissue with the same characteristics of native tendon. Actually, even surgical repair fails in to regenerate the characteristics of tendon or TBi, resulting in a fibrous healing tissue that leads to inferior biomechanical properties, thereby increasing the likelihood of recurrence of the tear (Carpenter et al. 1998; Kovacevic and Rodeo 2008; Lui 2015; Rodeo et al. 2007). For example, in rotator cuff’s TBi healing, instead of four zones, a three-layer fibrovascular construct is formed that has significantly less fibrocartilage than normal (Huegel et al. 2015), and specifically, the zone of mineralized fibrocartilage does not reform (Gerber et al. 1999).
13.4 Scaffolds and Biomaterials
TE using biomaterials is the focus of intense investigation worldwide and will probably be one of the treatments of choice for tendon and ligament injuries. It involves the use of cells, biomaterials, growth factors, or their combinations with the aim of directing a more sophisticated healing response to promote tissue repair.
TE strategies with cells require an adequate scaffold that could contain them and where cells can adhere, interact with specific growth factors and cytokines, proliferate, differentiate, and produce extracellular matrix for the success of cell therapy procedures (Krampera et al. 2006). Moreover, it provides mechanical support and may have biological properties that may favorably influence tendon-to-bone healing (Ladermann et al. 2015). Tissue regeneration through TE techniques can be achieved by culturing isolated cells on 3D scaffolds to develop biological substitutes, and for that it is important to increase the number of isolated cells (Shimode et al. 2007). Cell adhesion to the scaffold depends on the interaction that is established in between the scaffold microstructure and the cell surface receptors denominated integrins (Mora et al. 2015).
More recently, it has been used scaffolds made of biodegradable synthetic materials, which, by providing nonpermanent support to cells, can serve as temporary matrices for tissue regeneration, progressively disappearing as the new tissue is formed (Lorbach et al. 2015). Furthermore, the development of 3D electrospun scaffolds which through their close structural resemblance to ECM provide morphological cues encouraging cell growth, the application of functional tissue engineering (FTE) in scaffold design to obtain scaffolds with adequate mechanical properties and the development of fabrication techniques and coating technologies that facilitate the integration of bioactive molecules (e.g., growth factors) with scaffolds (Breidenbach et al. 2014; Hakimi et al. 2012).
13.4.1 Basic Considerations and Scaffold Design
Depending on the type of the biomaterial used, scaffolds are divided in synthetic, natural, or composite. There are several requirements, which should be met when designing scaffolds for tendon TE. The material should be biocompatible, promoting new tissue formation without immunological response. Sufficient void space is an important requirement in order to allow adequate nutrient delivery to the seeded or infiltrated cell population (Hollister 2005; Petrigliano et al. 2006). In fact this void space quantified as scaffold porosity is determinant for cellular proliferation and ECM formation, which enables the scaffold integration with host tissue (Hollister 2005; Laurencin and Freeman 2005; Vunjak-Novakovic et al. 2004). The goal is to achieve the same mechanical properties (e.g., elastic modulus, toughness, and ultimate strength) as the host tissue to restore normal physiologic function and prevent stress shielding of the newly formed tissue (Laurencin and Freeman 2005; Vunjak-Novakovic et al. 2004; Woo et al. 1999, 2006).
Moreover, biodegradability is an important feature. When implanting a scaffold, it is expected that this will provide the conditions for new tissue formation at the same rate of its own degradation that occurs (Doroski et al. 2007; Laurencin and Freeman 2005; Vunjak-Novakovic et al. 2004). Synthetic scaffolds offer the advantage of being able to be tuned to have a wider variety of mechanical properties and degradation characteristics through different fabrication and processing techniques. Despite these advantages, their degradation and wear products may cause an unwanted host immune response or toxicity. Natural scaffolds do not carry these risks and are readily degraded. The most popular current technique for production of nanofibrous scaffolds is electrospinning because it is a continuous, scalable process, which allows production of fibers ranging from nanometers to microns in either a random or aligned fashion (Fig. 13.3).


Fig. 13.3
Scanning electron microscopic images of (a) randomly oriented and (b) aligned keratin fibers fabricated by electrospinning. Scale bars represent 5 μm
13.4.2 Synthetic Scaffolds
Synthetic polymers allow precise control over molecular weight, degradation time, hydrophobicity, and other attributes, yet they may not interact with cells in a desired manner (Langer and Vacanti 1993).
First-generation synthetic scaffolds were inert materials, which failed to simulate the biomechanical properties of native tendon. They also did not behave as expected concerning creep, permanent deformation, stress shielding, abrasive wear and degradation, axial splitting, and extensibility (Petrigliano et al. 2006; Vunjak-Novakovic et al. 2004). Unlike the previous generation, new biomaterials have high initial strength and are designed to gradually transfer the load to the newly formed tissue, while the degradation process is progressing and the new tissue matures.
Poly(urethane) is a very heterogeneous and versatile group of polymers in what concerns their physical and mechanical properties. Depending on the fabrication process, these scaffolds can have different porosities and tunable degradation characteristics (Webb et al. 2006). These scaffolds seeded with fibroblasts have shown increased cell proliferation, matrix accumulation, and elastic modulus after a cyclic strain regimen (Webb et al. 2006). However, poly(urethanes) may release toxic degradation products and are unable to maintain similar mechanical properties to the native tendon upon degradation (Guelcher 2008).
Poly(esters) when used for tendon regeneration as scaffolds are produced in a fibrous form that is posteriorly processed to form higher ordered structures such as sheets (Deng et al. 2009; Wang et al. 2008), woven or knitted meshes (Heckmann et al. 2008), and braids (Freeman et al. 2007, 2009). PLLA, PGA, and PLGA are the most commonly used poly(esters) for tendon tissue engineering. The large interfiber pores have been shown to allow significant collagen deposition both in vitro and in vivo in a rabbit model for ACL reconstruction (Freeman et al. 2007, 2009; Van Eijk et al. 2008).
These materials have a wide range of degradation profiles and mechanical properties. However, a material that retains these properties during the replacement process by new tissue remains to be found (Vieira et al. 2009).
13.4.3 Natural Scaffolds
Also natural scaffolds have evolved during time. Initially, porous scaffolds composed of randomly oriented collagen type I fibers were used. These scaffolds without cells had suboptimal mechanical properties (Torres et al. 2000). In order to overcome this limitation, other approaches were tried. Gels composed of aligned collagen fibers seeded with MSCs and submitted to mechanical stimulation had showed significantly better mechanical properties (Altman et al. 2002; Shearn et al. 2007). MSC-seeded porous collagen sponges which generate aligned collagen fibers under cyclic strain have demonstrated increased ECM gene expression and protein deposition (Juncosa-Melvin et al. 2007). The main drawbacks of collagen matrices are the need for processing the collagen derived from animal tissue in order to remove foreign antigens and potential donor pathogens to reduce immunogenicity. These processes reduce the mechanical strength and collagen also undergoes fast in vivo degradation (Liao et al. 2008; Vieira et al. 2009).
Fibrin matrix cross-linked with thrombin and containing bone marrow stromal cells showed initially promising results, but as with collagen matrices, they are still unable to achieve the ideal mechanical properties (Liao et al. 2008; Vieira et al. 2009).
From the natural scaffolds discussed here, silk represents the strongest, being also biocompatible, promoting cell adhesion, proliferation, and ECM deposition (Vunjak-Novakovic et al. 2004). Its degradation occurs at a relative slow rate in vivo, and when its fibers are arranged in a wire rope, it achieves similar mechanical properties to those of native ligament or tendon (Chen et al. 2003, 2006; Moreau et al. 2008). However, silk presents some concerns about the adequate removal of contaminating proteins and potential immunogenicity since it is not a human native protein (Vieira et al. 2009).
13.4.4 Composite Scaffolds
Composite scaffolds are the result of the association of natural and synthetic materials in an attempt to use the positive aspects of each material in synergy. There are several studies in which fibrous scaffolds were combined with hydrogels to allow homogeneous cell seeding within the fibrous scaffold, promote the alignment of cells and ECM in the gel, reinforce the gel scaffold, and allow delivery of nutrients and growth factors through the gel (Fan et al. 2008; Hayami et al. 2010).
Some groups have been developing composite materials in order to replicate tendon interfaces. A stratified scaffold using three distinct continuous phases was produced using knitted meshes and sintered microspheres. Each phase was designed to reproduce a specific region of the tissue. This scaffold supported cell proliferation, migration, and production of ECM, maintaining distinct cellular regions and phase-specific ECM in vitro (Spalazzi et al. 2008).
Other approaches have been exploring the incorporation of ceramics with different fabrication technics to achieve graded mineral deposition (Paxton et al. 2008).
Human hair keratin offers great possibilities for tissue regeneration and repair applications because of its abundance, bioactive properties, and potential as a reliable autologous material (Rouse and Van Dyke 2010). Among existing biomaterials, fibrous matrices produced by electrospinning technique are widely used because they can mimic the nanoscale structure and complexity of the ECM and are controllable in fiber diameter and alignment (Khorshidi et al. 2016). It has been previously reported success in electrospinning randomly oriented keratin fibers by blending human hair keratin with a small amount of poly(ethylene oxide) (PEO) and showed that these are compliant for fibroblast attachment and proliferation (Sow et al. 2013).
Despite these scaffolds have an enormous potential, they are still in a premature phase of development. Their use in large scale for tendon regeneration in humans is still far from being a reality.
13.5 Tissue Engineering Strategies
The possible application of several cell types used in conjunction with biomaterials in order to improve tendon regeneration has been vastly studied. Biomaterials might be used in order to facilitate integration and recruitment of these cells from host tissue or in alternative cells can be seeded in biomaterials prior to implantation. The most promising cells for tendon regeneration are tenocytes, dermal fibroblasts, mesenchymal stem cells (MSCs), and tendon-derived stem cells (TDSCs).
13.5.1 Tenocytes
Once tenocytes are the predominant cell type found in native tendon, it is logical to integrate them in biomaterials matrices in order to try replicating native tendon. In fact, these cells are responsible for producing and maintaining the ECM which provides mechanical strength (Benjamin and Ralphs 2000). Tenocytes not only are readily incorporated into most scaffolds where they adhere and proliferate (Guelcher et al. 2008; Wang et al. 2008) but also native cells can undergo migration and infiltration into implanted scaffolds (Freeman et al. 2009). A considerable flaw in this process is the need for autogenous cells in order to avoid host immune response. These cells need to be harvested from the patient’s tendons, which may lead to significant donor site morbidity.
13.5.2 Dermal Fibroblasts
Dermal fibroblasts represent a good alternative in order to overcome the difficulties and complications associated to tendon fibroblast harvesting. These cells can be obtained through a simple skin biopsy (Cornwell et al. 2004, 2007). Concerning basic behavioral characteristics, these cells adhere and proliferate in a similar way and appear to synthesize many of the same ECM components (Deng et al. 2009). Not being derived from the same tissue for which they are being applied remains the question if at long term the behavior will be similar to that of native tendon.
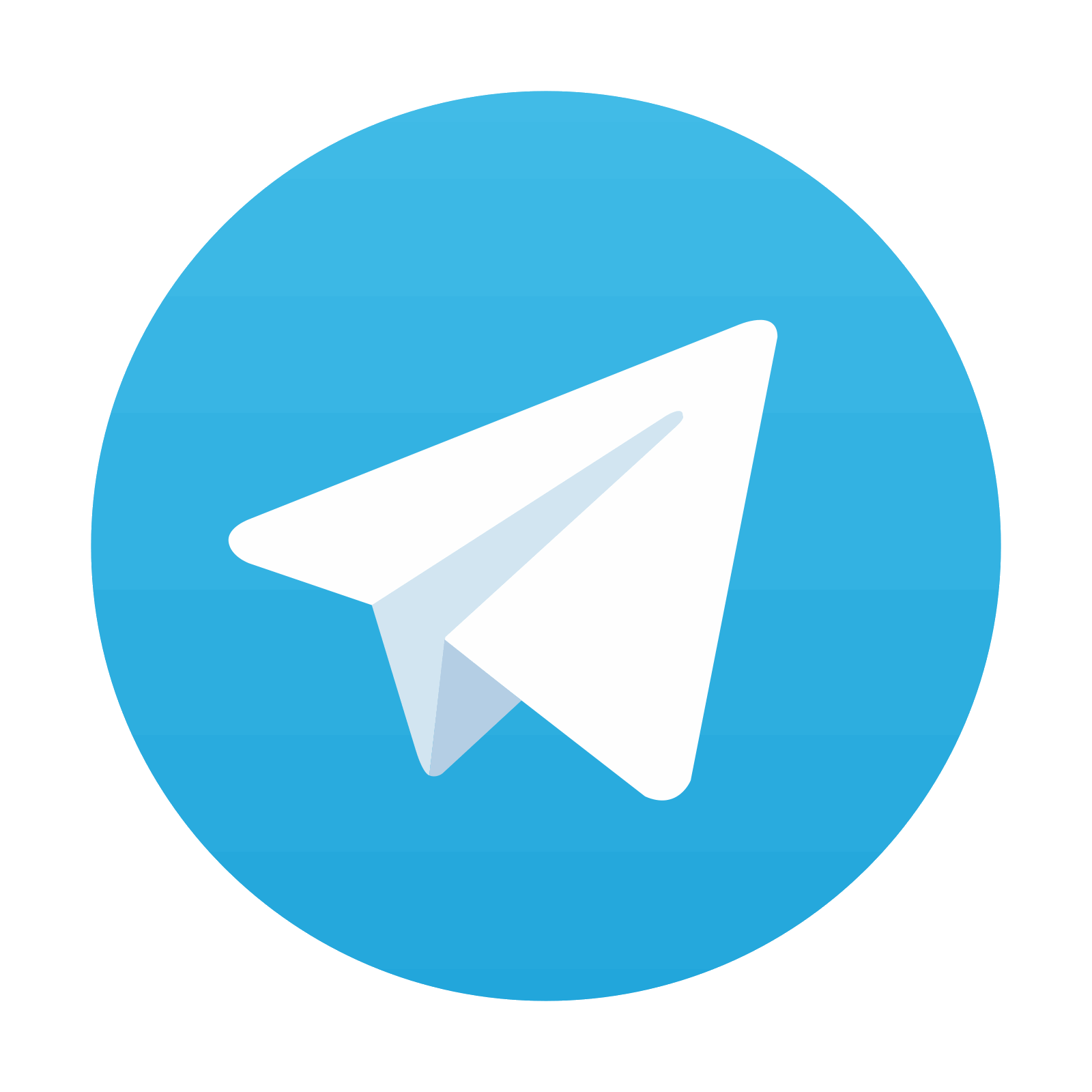
Stay updated, free articles. Join our Telegram channel
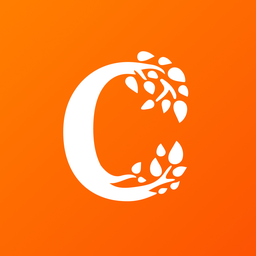
Full access? Get Clinical Tree
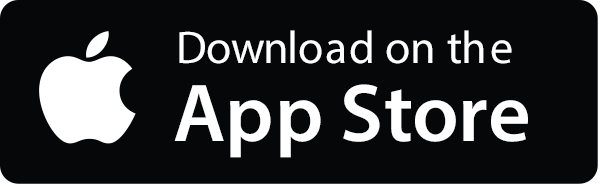
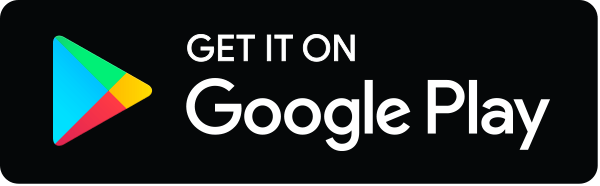