Chapter 1 Basic Science of Ligaments and Tendons Related to Rehabilitation
After studying this chapter, the reader will be able to do the following:
Ligaments and tendons traverse diarthrodial joints to provide stability and to mediate normal joint motion. The structure and composition of ligaments and tendons give them the flexibility and tensile strength required to perform their functions. Tendons connect muscles to bones and transmit muscle forces to generate joint movement. Tendons also absorb shock to prevent damage to the muscle. Ligaments help to position the bones in appropriate alignment for muscle action and also guide joint motion. In the case of excessive external loading, the stiffness of the ligament would increase and thereby limit excessive displacements between bones so that joint loads would not damage soft tissues, such as the articular cartilage and meniscus. Because of their respective functions, ligaments and tendons display slight differences in their histomorphologies, biochemical compositions, biomechanical properties, and intrinsic healing response. Furthermore, biological factors, such as age, homeostatic responses secondary to immobilization or mobilization of the joint, tension, and exercise, can affect their properties.1–5
Injuries to ligaments and tendons resulting from excessive forces or overuse can disrupt the balance between mobility and joint stability, resulting in damage to soft tissues in and around the joint that leads to pain, morbidity, and even osteoarthritis. The incidence of ligament and tendon injuries in the United States has reached epidemic proportions and affects daily function in both occupational and athletic settings. Ligaments, particularly the knee ligaments, are especially susceptible to injury during sports activities. As many as 150,000 new anterior cruciate ligament (ACL) injuries occur annually in the United States.6 In addition, approximately 100,000 medial collateral ligament (MCL) and 25,000 posterior cruciate ligament injuries occur annually.7 The incidence of tendon injuries has also increased over the past few decades. For example, tendinopathy accounts for an estimated 30% to 50% of all sports injuries.8
Treatment of these injuries ranges from rest or passive immobilization for MCL and some tendon injuries to surgical reconstruction for ACL injuries using tendon autografts and allografts. These treatment options vary on the basis of the ligament or tendon’s intrinsic ability to heal or lack of it. However, current treatment modalities have been shown to result in healing tissues that have inferior properties and cannot entirely restore normal joint function.9,10 Hence the basic science behind ligament and tendon function, injury mechanism, and healing process needs to be better understood so that treatment strategies can be developed.
BASIC SCIENCE
Biology and Biochemical Composition
Ligaments and tendons have a highly organized extracellular matrix (ECM) of densely packed collagen fiber bundles. The alignment of these collagen fiber bundles generally follows the lines of tension applied to the ligament or tendon. These bundles are composed of tropocollagen as the basic molecular component. Tropocollagen molecules are systematically arranged into a hierarchic structure of microfibrils, subfibrils, fibrils, and fibers (Figure 1-1, A).11 Inspection of ligaments and tendons under polarized light microscopy reveals that the arrangement of collagen fibrils within the bundles has a sinusoidal wave or crimp pattern that can elongate easily when a force is applied (Figure 1-1, B). When the force is removed, the fibers recoil and return to the original crimp pattern configuration.
Insertions of ligaments and tendons into the bone consist of transitional zones from soft to hard tissues in order to minimize stress concentrations. Morphologically, these insertions can be direct or indirect. In a direct insertion, there are four distinct zones of transition: ligament or tendon, uncalcified fibrocartilage, calcified fibrocartilage, and finally bone (Figure 1-2, A).12,13 In indirect insertions the superficial layers of the ligament or tendon connect with the periosteum, whereas the deeper layers connect directly to bone via Sharpey’s fibers (Figure 1-2, B).12,13 The MCL of the knee is an example of a ligament that exhibits both types of insertions (i.e., its femoral insertion is direct while its tibial insertion is indirect).
Ligaments and tendons are relatively hypocellular, with fibroblasts interspersed throughout the tissue matrix. Fibroblasts are responsible for the maintenance and remodeling of the ECM through the secretion of macromolecules, such as collagen, elastin, and proteoglycans.14 Collagen type I (70% to 90% of the dry weight) is the main component of ligaments and tendons and is the main contributor to their tensile stiffness and strength. Many other collagen types, such as III, V, IX, X, XI, and XII, also exist, but only in lesser amounts. Although the significance of some of these minor collagen types is not completely clear, some recent findings have helped to elucidate their roles within the tissue. For example, type V collagen is believed to exist in association with type I collagen and serves as a regulator of collagen fibril diameter,15,16 whereas type III collagen is thought to be elevated during healing.17 Type XIV collagen provides lubrication between collagen fibers.18 Collagen has the ability to form covalent intramolecular (aldol) and intermolecular (Schiff base) cross-links, which are key contributors to its tensile strength and resistance to chemical or enzymatic breakdown.19
Elastin, another fibrous protein present in ligaments and tendons (usually <1% of the dry weight), allows the tissue to return to its prestretched length following physiological loading. Other constituents include proteoglycans and glycosaminoglycans. They form only a small portion of the macromolecular framework of the ligament (<1%), but they have a significant role in the formation and organization of the ECM. The chemical structure, intermolecular cross-linking of the collagen, and its interaction with other ECM macromolecules bring a hydrophilic nature to maintain the high water content (65% to 70% of the total weight) that is crucial to the unique function of these tissues.14
Biomechanics
Because ligaments and tendons function to resist tensile loads, uniaxial tensile tests are performed on these tissues to characterize their biomechanical behavior, as well as their relative contribution to joint kinematics. Generally, a bone-ligament-bone complex or bone-tendon-muscle complex is tested, and the resulting load-elongation curve exhibits nonlinearity that has an initial nonlinear region called the “toe region” of lower stiffness. With further loading, the curve gradually stiffens into a “linear region” (where the slope of the curve becomes constant). Finally, continuous increase in loading will eventually cause the specimen to fail (Figure 1-3, A). The load-elongation curves represent the tensile behavior of the whole structure including bony or muscle insertions, or both (i.e., to mimic ligament or tendon function in vivo). Parameters representing the structural properties (load-elongation curve) including stiffness, ultimate load, ultimate elongation, and energy absorbed at failure, are obtained. The definitions of these parameters (see Figure 1-3, A) are listed as follows:
To assess the quality of a ligament or tendon, a stress-strain curve representing the mechanical properties must be obtained so that the properties of different tissues can be compared. Mechanical properties yield a better description of the effects of biological factors and mechanical factors on the quality of the tissues. The stresses and strains of a defined region of the ligament or tendon substance can be calculated from a uniaxial tensile test, as described previously. Stresses can be obtained by normalizing the load with respect to the cross-sectional area whereas strain is measured on the basis of the change in length of the specimen with respect to the original (gauge) length of the specimen. To perform these measurements, it is best to determine the cross-sectional area of the specimen by using a laser micrometer system.20,21 For strain, video cameras and a video dimensional analysis system are used to track the position of reflective surface markers placed on the ligament.22 Thus measuring stress and strain is possible without making physical contact with these soft tissues. From the stress-strain curves, the parameters representing mechanical properties including tangent modulus, tensile strength, ultimate strain, and strain energy density of the ligament or tendon substance, are obtained. The definitions of these parameters (Figure 1-3, B) are listed as follows:
Ligaments and tendons also display time- and history-dependent viscoelastic properties that reflect the complex interactions among collagen, elastin, proteoglycans, and water.23,24 For example, the loading and unloading curves of these tissues do not follow the same path but instead form a hysteresis loop representing internal energy dissipation following a cycle of loading and unloading (Figure 1-4). However, over the course of several cycles, the area of hysteresis is reduced, and the curves become more repeatable. Because the mechanical behavior of a tissue tested after the first cycle will differ from that of a tissue tested at the tenth cycle, the specimen must be preconditioned by a number of cycles to obtain more consistent data. Other important viscoelastic characteristics of ligaments and tendons include creep, an increase in deformation over time under a constant load or stress, and stress relaxation, a decline in stress over time under a constant deformation or stress (Figure 1-5).
The viscoelastic behavior of ligaments and tendons has important clinical significance. For example, during walking or jogging, cyclic stress relaxation decreases a tissue’s resistance to strain, resulting in a continuous decrease in peak stress for each cycle.14,25 This phenomenon may help prevent fatigue failure of ligaments and tendons. Similarly, cyclic creep can be used to demonstrate how warm-up exercises and stretching can increase flexibility of a joint as a constant applied stress during stretching increases the length of the ligaments and tendons.
Tissue Homeostasis
Ligaments and tendons respond to physical stimuli in vivo and undergo changes accordingly. A generalized statement similar to Wolff’s law26 for bone can be made for ligaments and tendons because they do respond to these physiological stress and motion through remodeling of their morphological appearance and biochemical constituents, as well as biomechanical properties. In the past, ligament injuries were treated with prolonged immobilization of joints followed by a delayed therapeutic exercise after surgery. In time, as the detrimental effects of immobilization were elucidated by laboratory and clinical studies, the clinical paradigm shifted toward the introduction of stress as rapidly as possible to limit the damage to the joint, as well as to improve its healing. On the other hand, overzealous therapeutic approaches that introduce excessive stress or motion can cause further injury and retard recovery. Hence observing the time course for immobilization and mobilization are important for an athlete or worker to effectively return to sport or work after suffering an injury. Any rehabilitation procedure begs the question of how soon and how much. The answers must be tailored to the age and physical state of the patient, as well as the location and severity of injury.
Immobilization
Immobilization of a joint induces concomitant stress deprivation of surrounding healthy structures, which can adversely affect them.14 For example, immobilization can cause intra-articular changes including pannus formation, which leads to necrosis and erosion and ulceration of the articular cartilage.27,28 Increased joint stiffness is also commonly observed clinically and has been demonstrated quantitatively in experimental animals.29,30 After 9 weeks of immobilization, the amount of torque required to initially extend a rabbit knee is significantly increased.29 Immobilization also causes the other tissues, such as joint capsule, to be cross-linked, which contributes to joint stiffness.29
In ligaments and tendons, alterations in collagen cross-linking, synthesis, and degradation occur following immobilization.31 These changes lead to decreased stiffness and ultimate load of a ligament or tendon complex (Figure 1-6). The ultimate loads and energy absorbed at failure of the femur-MCL-tibia complexes (FMTC) are only 31% and 18%, respectively, of the contralateral nonimmobilized controls.13 The tangent modulus of the MCL substance is significantly decreased, revealing a softening of the ligament substance after immobilization. Failures occur at the tibial insertion rather than at the midsubstance because of increasing osteoclastic activities at the insertion sites.32 Different ligaments such as the ACL experience fewer changes in tensile properties than the MCL of the same knee after 9 weeks of immobilization.33 Therefore it appears that different soft tissue structures are affected differently by immobilization, and care must be exercised when generalizing specific results to all ligaments or tendons.
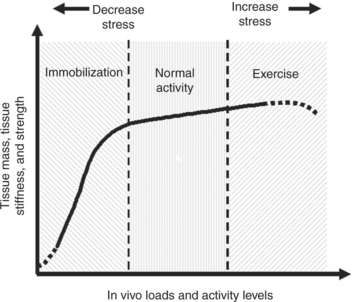
Figure 1-6 Schematic diagram illustrating ligament homeostatis secondary to stress and motion.
(From Woo SL, Chan SS, Yamaji T: J Biomechics 30(5):431-439, 1997.)
Remobilization of the knee joint following immobilization reveals that the mechanical properties of the MCL substance in the functional range return to normal values relatively rapidly.13 The mode of failure for the remobilized limb continues to occur by disruption at the bony insertion sites, suggesting recovery at these sites remains incomplete. These findings imply an asynchronous rate of recovery between the ligament and bony insertion, which has strong implications for how to develop appropriate rehabilitation strategies.34
Exercise
The effects of exercise can have specific influence on different ligaments and tendons. In a study of the effect of short-term (3 months) and long-term (12 months) exercise on the biomechanical properties of swine digital tendons, short-term exercise demonstrated little or no effect on the digital extensor tendon properties, whereas long-term exercise resulted in positive changes including an increase in cross-sectional area, as well as a 22% increase in tensile strength.35,36 On the other hand, for the digital flexor tendons, the mechanical properties of the tendon substance exhibited no statistical changes for both short-term and long-term exercise, whereas the ultimate load of the exercised flexor-tendon complexes increased by 6% in the short-term exercised group and 19% in the long-term exercised group secondary to changes at the bony insertion sites.5
On the basis of these findings, a spectrum may exist for tissue response to different activity levels to maintain homeostasis. Figure 1-7 shows a hypothetical curve that describes the homeostatic responses for soft tissues, such as ligaments and tendons. The relationship between the level and duration of stress and motion and the in vivo biological responses in tissue properties and tissue mass is represented by a highly nonlinear curve. With stress and motion deprivation (immobilization), a rapid reduction in tissue properties and mass occurs. In contrast, the gains following exercise training are much less pronounced. Experts also know that the functional integrity of an immobilized ligament can return to its normal mechanical characteristics quite rapidly following remobilization.13 However, the recovery of the ligament-bone junction is much slower than that of the ligament substance.
Effects of Aging
Biomechanical changes associated with skeletal maturation and senescence have been documented in the MCL, ACL, and patellar tendon using animal models, as well as human cadavers.1,2,37,38 These studies show that different soft tissue structures are affected differently by aging. Significant increases in the linear stiffness, ultimate load, and energy absorbed at failure of the FMTC were noted in both the male and female rabbits during skeletal maturation. Afterward, only slight decreases in these properties were found with increasing age. The modes of failure correlated well with closure of the epiphyses in both sexes (i.e., tibial avulsion failure for the skeletally immature groups and ligament midsubstance for the skeletally mature groups). The tangent modulus of the rabbit MCL substance increased during maturation for both sexes until 12 months of age and then gradually declined until 48 months. The ultimate tensile strength also remained relatively constant after 12 months of age and reduced slightly at 48 months. Thus the rate of skeletal maturation is a significant contributor to the differences in the tensile properties of both the male and female rabbits.39
In the case of ACL, data on age-related changes have been obtained from human cadavers. In a study using pairs of human cadaveric knees obtained from young donors (22 to41 years, mean age 35 years) and older donors (60 to 97 years, mean age 76 years), there was a rapid decline in the stiffness and ultimate load in the femur-ACL-tibia complex as a function of age.2 These differences in the biomechanical properties for older individuals may be due to a decrease in the level of activity with advancing age, the changes in hormone levels, and other physiological or geometric changes of the joints.
For the rabbit patellar tendon, a decrease in maximum stress was found between 1- and 4-year-old rabbits whereas minimal differences were found for other biomechanical parameters.37 The age-related changes in tensile and viscoelastic properties were investigated using fresh-frozen human patellar tendons. Specimens for the younger group were from individuals 29 to 50 years of age, whereas those from the older group were from individuals 64 to 93 years of age. Except for ultimate tensile strength, which was 17% less for the older group than the younger group, minimal differences in tensile and viscoelastic properties existed between younger and older groups.38 Another study evaluated the biomechanical properties of patellar tendon allografts from donors aged 18 to 55 years. There was no significant correlation between age and any of the mechanical properties.40 The data from these studies imply that patellar tendon allografts harvested from individuals up to 55 years of age can be used for ACL reconstruction without a significant loss in the mechanical properties.
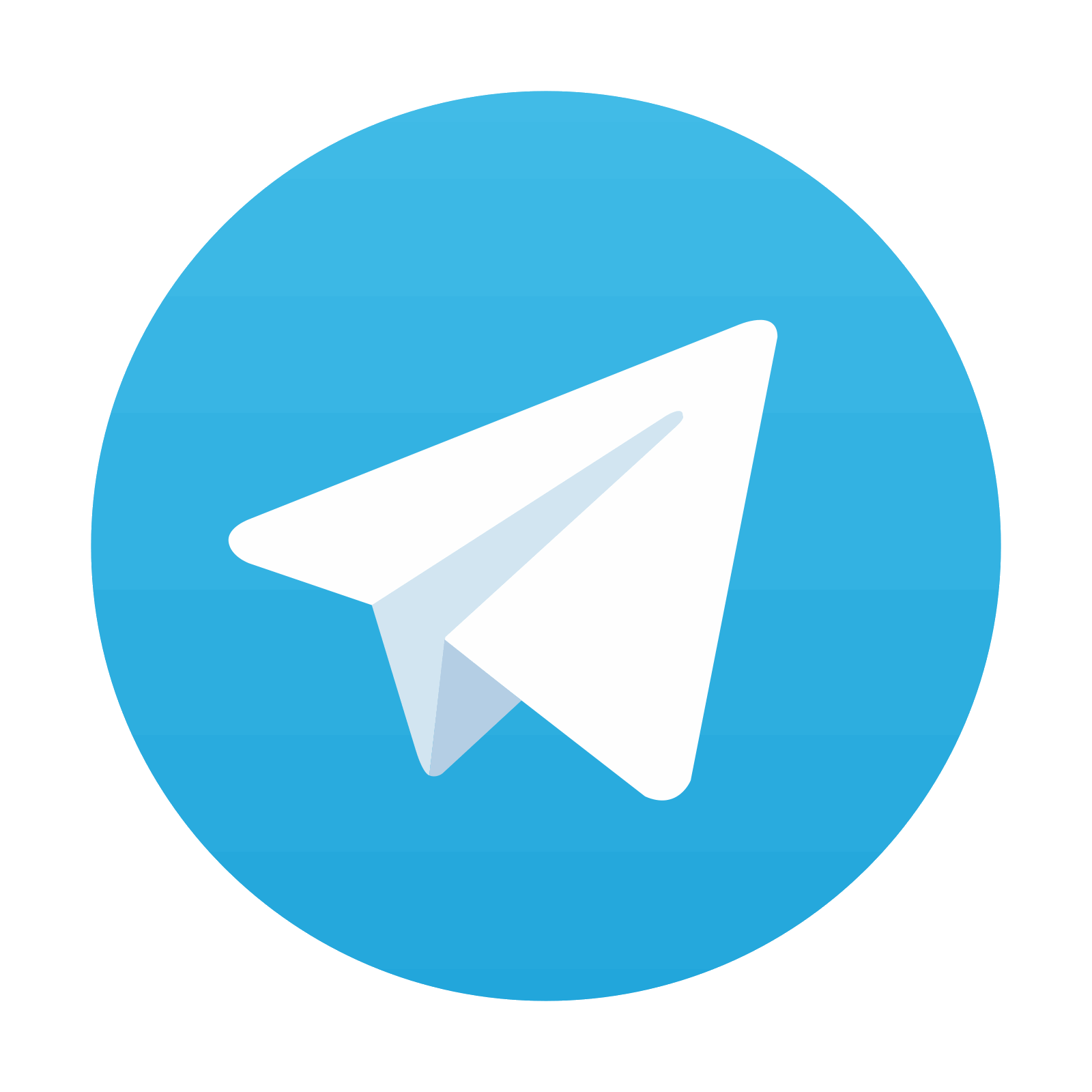
Stay updated, free articles. Join our Telegram channel
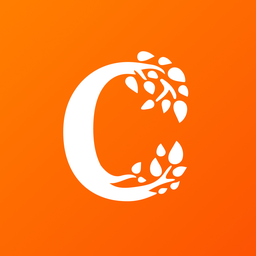
Full access? Get Clinical Tree
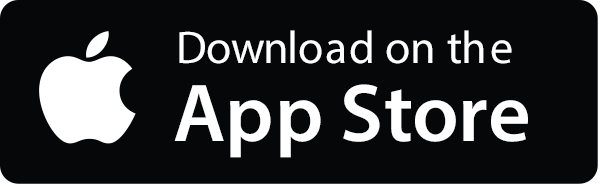
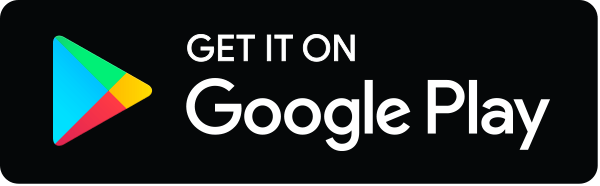