Chapter 1 César Fernández-de-las-Peñas; Jan Dommerholt Myofascial trigger points (TrPs) are one of the most overlooked and ignored causes of acute and chronic pain (Hendler & Kozikowski, 1993). At the same time, TrPs constitute one of the most common musculoskeletal pain conditions (Hidalgo-Lozano et al., 2010; Bron et al., 2011a). There is overwhelming evidence that muscle pain is commonly a primary dysfunction (Mense, 2010a) and not necessarily secondary to other diagnoses. Muscles feature many types of nociceptors, which can be activated by a variety of mechanical and chemical means (Mense, 2009). As a primary problem, TrPs may occur in absence of other medical issues; however, TrPs can also be associated with underlying medical conditions (e.g., systemic diseases) or certain metabolic, parasitical, and nutritional disorders. As a comorbid condition, TrPs can be associated with other conditions such as osteoarthritis of the shoulder, hip, or knee (Bajaj et al., 2001) as well as with injuries such as whiplash (Freeman et al., 2009). Pain elicited by muscle TrPs constitutes a separate and independent cause of acute and especially chronic pain that may compound the symptoms of other conditions and persist long after the original initiating condition has been resolved. TrPs are also associated with visceral conditions and dysfunctions, including endometriosis, interstitial cystitis, irritable bowel syndrome, dysmenorrhea, and prostatitis (Weiss, 2001; Anderson, 2002; Doggweiler-Wiygul, 2004; Jarrell, 2004). The presence of abdominal TrPs was 90% predictive of endometriosis (Jarrell, 2004). Throughout history TrPs have been referred to by different names (Simons, 1975). The current TrP terminology has evolved during the past several decades (Simons et al., 1999; Dommerholt et al., 2006; Dommerholt & Shah, 2010). Although different definitions of TrPs are used among different disciplines, the most commonly accepted definition maintains that ‘a TrP is a hyperirritable spot in a taut band of a skeletal muscle that is painful on compression, stretch, overload, or contraction of the tissue, which usually responds with a referred pain that is perceived distant from the spot’ (Simons et al., 1999). From a clinical viewpoint, we can distinguish active and latent TrPs. The local and referred pain from active TrPs reproduces the symptoms reported by patients and is recognised by patients as their usual or familiar pain (Simons et al., 1999). Both active and latent TrPs cause allodynia at the TrP and secondary hyperalgesia after applied pressure. Allodynia is pain due to a stimulus that does not normally provoke pain. In latent muscle TrPs the local and referred pain do not reproduce any symptoms familiar or usual to the patient (Simons et al., 1999). Active and latent TrPs have similar physical findings. The difference is that latent TrPs do not reproduce any spontaneous symptoms other than referred pain. In patients with lateral epicondylalgia, active TrPs commonly reproduce the symptoms within the affected arm (Fernández-Carnero et al., 2007); however, patients may also present with latent TrPs on the nonaffected side without experiencing any symptoms on that side (Fernández-Carnero et al., 2008). In a recent Delphi study with 60 experts from 12 countries, 80% of the participants agreed that referred pain from TrPs can include different sensory sensations, including pain, a dull ache, tingling, or burning pain. Eighty-four percent of the experts agreed that the reproduction of symptoms experienced by patients and the recognition of pain are the main clinical differences between active and latent TrPs (Fernández-de-las-Peñas & Dommerholt, 2018). Although latent TrPs are not spontaneously painful, they provide nociceptive input into the dorsal horn (Ge et al., 2008, 2009; Li et al., 2009; Zhang et al., 2009; Wang et al., 2010; Xu et al., 2010; Zhang et al., 2009; Ge & Arendt-Nielsen, 2011). The underlying mechanism is not clear at this point in time and requires more research. Certain regions within a muscle may only be connected via ineffective synapses to dorsal horn neurons and referred pain may occur when these ineffective synapses are sensitised (Mense, 2010b). Latent TrPs can easily turn into active TrPs, which is at least partially dependent on the degree of sensitisation and increased synaptic efficacy in the dorsal horn. For example, the pain pressure threshold of latent TrPs in the forearm muscles decreased significantly after only 20 minutes of continuous piano playing (Chen et al., 2000). Active TrPs induce larger referred pain areas and higher pain intensities than latent TrPs (Hong et al., 1997). Active TrPs and their overlying cutaneous and subcutaneous tissues are usually more sensitive to pressure and electrical stimulation than latent TrPs (Vecchiet et al., 1990, 1994). Both active and latent TrPs can provoke motor dysfunctions, for example, muscle weakness, inhibition, increased motor irritability, spasm, muscle imbalance, and altered motor recruitment (Lucas et al., 2004, 2010) in either the affected muscle or in functionally related muscles (Simons et al., 1999). Lucas and colleagues (2010) demonstrated that latent TrPs were associated with impaired motor activation pattern, confirmed more recently by Bohlooli and colleagues (2016). Elimination of these latent TrPs induces normalisation of the impaired motor activation pattern (Lucas et al., 2010). In another study, restrictions in ankle range of motion were corrected after manual release of latent TrPs in the soleus muscle (Grieve et al., 2011). Manual treatment of latent TrPs in the upper trapezius muscle significantly decreased TrP sensitivity, increased flexibility of muscle fibres, and improved range of motion (Ganesh et al., 2016; Kojidi et al., 2016). Referred pain is a phenomenon described for more than a century and has been used extensively as a diagnostic tool in the clinical setting. Typically pain from deep structures such as muscles, joints, ligaments, tendons, and viscera is described as deep, diffuse, and difficult to locate accurately in contrast to superficial types of pain such as pain originating in the skin (Mense, 1994). Pain located at the source of pain is termed local pain or primary pain, whereas pain felt in a different region away from the source of pain is termed referred pain or secondary hyperalgesia (Ballantyne et al., 2010). Referred pain can be perceived in any region of the body, but the size of the referred pain area is variable and can be influenced by pain-induced changes in central somatosensory maps (Kellgren, 1938; Gandevia & Phegan, 1999). Referred pain is a very common phenomenon in clinical practice; most patients with chronic pain present with what is commonly described as ‘a summation of referred pain from several different structures‘. Understanding at least the basic neurophysiological mechanisms of muscle referred pain is required to make a proper diagnosis of myofascial pain and to manage patients with TrPs. Clinical characteristics of muscle referred pain (Arendt-Nielsen & Ge, 2009; Fernández-de-las-Peñas et al., 2011) include the following: The exact neuropathways mediating referred pain are not completely understood, but there are enough data to support that ‘muscle referred pain is a process of central sensitisation, which is mediated by a peripheral activity and sensitisation, and which can be facilitated by sympathetic activity and dysfunctional descending inhibition‘ (Ge et al., 2006; Arendt-Nielsen & Ge, 2009). Increased autonomic activity is likely due to activation of adrenergic receptors at the motor endplate (Gerwin et al., 2004). Stimulation of these adrenergic receptors triggered an increased release of ACh in mice (Bowman et al., 1988). Nociceptive stimuli of latent TrPs can lead to autonomic changes such as vasoconstriction (Kimura et al., 2009). The local or systemic administration of the alpha-adrenergic antagonist phentolamine to TrPs caused an immediate reduction in electrical activity, suggesting that TrPs indeed have an autonomic component (Hubbard & Berkoff, 1993; Lewis et al., 1994; McNulty et al., 1994; Banks et al., 1998). Such increased autonomic activity may facilitate an increased concentration of intracellular ionised calcium and be responsible again for a vicious cycle maintaining TrPs (Gerwin et al., 2004; Gerwin, 2008). Muscle spindle afferents may also contribute to the formation of TrP taut bands via afferent signals to extrafusal motor units through H-reflex pathways (Ge et al., 2009) but are not considered to be the primary cause of TrP formation. Several neuroanatomical and neurophysiological theories regarding the appearance of referred pain have been suggested. All models agree that nociceptive dorsal horn or brainstem neurons receive convergent inputs from different tissues. Consequently, higher brain centres cannot identify the input source properly. Most recent models have included newer theories in which sensitisation of dorsal horn and brainstem neurons also plays a relevant and central role. Following is a brief summary the most common theories. Ruch (1961) proposed that afferent fibres from different tissues such as skin, viscera, muscles, and joints converge onto common spinal neurons, which can lead to a misinterpretation of the source of nociceptive activity from the spinal cord. The source of pain of one tissue can be misinterpreted as originating from other structures. The convergent-projection theory would explain the segmental nature of muscle referred pain and the increased referred pain intensity when local pain is intensified. This theory does not explain, however, the delay in the development of referred pain after the onset of local pain (Graven-Nielsen et al., 1997a). The somatosensory sensitivity changes reported in referred pain areas can in part be explained by sensitisation mechanisms in the dorsal horn and brainstem neurons, whereas the delay in appearance of referred pain can be explained because the creation of central sensitisation needs time (Graven-Nielsen et al., 1997a). Bifurcation of afferents from different tissues was suggested as an explanation of referred pain (Sinclair et al., 1948). Although bifurcation of nociceptive afferents from different tissues exits, it is generally agreed that these pathways are unlikely to occur (McMahon, 1994). The axon-reflex theory cannot explain the delay in the appearance of the referred pain, the different thresholds required for eliciting local pain versus referred pain, or the somatosensory sensitivity changes within the referred pain areas. Theobald (1949) suggested that referred pain appears as a summation of input from the injured area and from the referred pain area within neurons in the brain but not in the spinal cord. Several decades later, Apkarian and colleagues (1995) described several pathways converging on different subcortical and cortical neurons. There is evidence of pain reduction after anaesthetisation of the referred pain area, which suggests that peripheral processes contribute to referred pain, although central processes are assumed to be the most predominant. Recordings from dorsal horn neurons in animal models have revealed that new receptive fields at a distance from the original receptive field emerged within minutes after noxious stimuli (Hoheisel et al., 1993): that is, after nociceptive input, dorsal horn neurons that were previously responsive to only one area within a muscle began to respond to nociception from areas that previously did not trigger a response. The appearance of new receptive fields could indicate that latent convergent afferents on the dorsal horn neuron are opened by noxious stimuli from muscle tissues (Mense, 1994) and that this facilitation of latent convergence connections induces the referred pain. More recently, similar patterns have been identified for nociceptive input from fascial structures (Taguchi et al., 2008). In fact, several studies showed that mechanical hyperalgesia was larger in fascia than in muscle (Schilder et al., 2014; Weinkauf et al., 2015). The central hyperexcitability theory is consistent with most of the characteristics of muscle and fascia referred pain. There is a dependency on the stimulus and a delay in appearance of referred pain compared with local pain. The development of referred pain in healthy subjects is generally distal and not proximal to the site of induced pain, (Arendt-Nielsen et al., 2000), although several clinical studies have demonstrated proximal and distal referred pain in patients with chronic pain (Graven-Nielsen, 2006). The differences between healthy individuals and persons with persistent pain may indicate that preexisting pain may induce a state of hyperexcitability in the spinal cord or brainstem, resulting in proximal and distal referred pain. TrPs are located within discrete bands of contractured muscle fibres, which are commonly referred to as taut bands in the TrP literature. Taut bands can be palpated with a flat or pincer palpation and feel like tense strings within the belly of the muscle. It is important to clarify that contractures are not the same as muscle spasms. Muscle spasms require electrogenic activity, meaning that the α-motor neuron and the neuromuscular endplate are active. A muscle spasm is a pathological involuntary electrogenic contraction (Simons & Mense, 1998). In contrast, a taut band signifies a contracture arising endogenously within a certain number of muscle fibres independent of electromyogenic activity, which does not involve the entire muscle (Simons & Mense, 1998). In 1997 Gerwin and Duranleau first described the visualisation of taut bands using sonography, but until recently it was not yet possible to visualise the actual TrP (Lewis & Tehan, 1999), mostly due to technological limitations (Park & Kwon, 2011). With the advancement of technology, more recent studies have found that TrP taut bands can be visualised using sonographic and magnetic resonance elastography (MRE) (Chen et al., 2007, Chen, Basford, & An et al., 2008, 2016; Sikdar et al., 2009; Bubnov, 2010; Rha et al., 2011; Maher et al., 2013; Müller et al., 2015). Chen and colleagues (2007) demonstrated that the stiffness of the taut bands in patients with TrPs is higher than that of the surrounding muscle tissue in the same subject and in people without TrPs. Sikdar and colleagues (2009) showed that vibration amplitudes assessed with spectral Doppler were 27% lower on average within the TrP region compared with surrounding tissue. They also found reduced vibration amplitude within the hypoechoeic region identified as a TrP. In summary, TrP taut bands are detectable and quantifiable, providing potentially useful tools for TrP diagnosis and future studies. Research to determine the correlation of clinician-identified myofascial taut bands with their presence and characteristics on MRE imaging showed that the agreement between physicians and MRE raters was relatively poor. The presence of taut bands as localised areas of increased muscle stiffness was confirmed once again, meaning they can be assessed quantitatively (Chen et al., 2016). Although TrPs and taut bands can now be visualised, the mechanism for the formation of muscle taut band is still not fully understood (Gerwin, 2008). The current thinking is that the development of the taut band and subsequent pain are related to local muscle overload or overuse when the muscle cannot respond adequately, particularly after unusual or excessive eccentric or concentric loading (Gerwin et al., 2004; Gerwin, 2008; Mense & Gerwin, 2010). Muscle failure and TrP formation are also common with submaximal muscle contractions as seen, for example, in the upper trapezius muscles of computer operators (Treaster et al., 2006; Hoyle et al., 2011) or in the forearm muscles of pianists (Chen et al., 2000). The failure of the muscle to respond to a particular acute or recurrent overload may be the result of a local energy crisis. Muscle activation in response to a demand is always dispersed throughout the muscle among fibres that are the first to be contracted and the last to relax. These fibres are the most vulnerable to muscle overload. Unusual or excessive eccentric loading may cause local muscle injury. In submaximal contractions, the Cinderella hypothesis and Henneman’s size principle apply (Kadefors et al., 1999; Chen et al., 2000; Hägg, 2003; Zennaro et al., 2003; Treaster et al., 2006; Hoyle et al., 2011). Smaller motor units are recruited first and derecruited last without any substitution of motor units. This would lead to local biochemical changes without muscle breakdown, especially in those parts of the muscle that are not substituted and therefore most heavily worked (Gerwin, 2008). Under normal physiological conditions, nerve impulses from a α-motor neuron reach the motor nerve terminal orthodromically and open voltage-gated sodium (Na+) channels, which trigger a Na+ influx that depolarises the terminal membrane. Next, voltage-gated P-type calcium (Ca2 +) channels are opened, causing an influx of Ca2 + and a quantal, but graded, release of approximately 100 acetylcholine-containing synaptic vesicles (ACh), adenosine triphosphate (ATP), serotonin (5HT), glutamate, and calcitonin gene-related peptide (CGRP), among other molecules from the nerve terminal into the synaptic cleft (Wessler, 1996; Malomouzh et al., 2007). The release of ACh is highly regulated; normally, inhibitory neuronal receptors, including muscarinic, alpha 2- and beta-adrenoceptors, nitric oxide (NO) receptors, and purinergic P2Y receptors, among others, prevent an excessive release of acetylcholine release (Wessler, 1996). Under normal circumstances, these inhibitory mechanisms prevent the development of persistent contractures as seen in myofascial pain. Of importance is that ACh release can be quantal and nonquantal. After exocytosis, ACh crosses the synaptic cleft and binds to acetylcholine receptors (AChR) on the motor endplate. In less than a millisecond, ACh is partially diffused and partially hydrolyzed by the enzyme acetylcholinesterase (AChE) into acetate and choline. Choline is reabsorbed into the nerve terminal where, by combining choline and acetyl coenzyme A from the mitochondria, it is synthesised into ACh via acetyltransferase. ACh release is modulated by the concentration of AChE. There are two sources of AChE in the synaptic cleft. A soluble form of AChE prevents ACh from reaching the receptors. The second source is the AChE that is found within the synaptic clefts; this AChE removes ACh from the receptors binding sites. Inhibition of AChE will cause an accumulation of ACh in the synaptic cleft, which may stimulate motor nerve endings and tonically activate nAChRs. Inhibition of AChE may also cause an increase of intracellular levels of Ca2 +, which likely contributes to the formation of taut bands. AChE is inhibited in an acidic environment and by calcitonin gene-related peptide. The nAChRs are temporarily inhibited after stimulation by ACh (Magleby & Pallotta, 1981). As a side note, ATP also plays a key role in the synthesis of AChE and nAChR through P2Y1 nucleotide receptors (Choi et al., 2003). The nonquantal release does not involve activation via the α-motor neuron. Nonquantal release contributes to maintaining the functional properties of skeletal muscles and to various neurotrophic functions of the motor endplate itself. It may also contribute to the formation of abnormal contractures or taut bands seen in myofascial pain conditions. TrPs are often viewed as loci of multiple localised sarcomere contractions, but this has not yet been fully confirmed. Biopsy studies of TrPs in fresh cadavers showed fully contracted sarcomeres with an absence of the I-band and an excess of the A-band (Reitinger et al., 1996; Windisch et al., 1999), but one cannot assume that no changes occurred after the time of death and before the onset of rigor mortis. Presynaptic ATP inhibits the release of quantal ACh through purinergic P2Y receptors, which implies that a decrease in ATP would lead to an increased release of ACh, which may be involved in the TrP pathophysiology. This process is also redox dependent, and, as such, oxidative stress may further facilitate the presynaptic inhibition. Of interest is that the nonquantal release of ACh is also significantly reduced by ATP, but not by adenosine. Blocking ATP with the purinergic receptor antagonist suramin ceases the effect of ATP and immediately increases the nonquantal secretion of ACh. Suramin also inhibits the activity of NO synthase, and a lack of NO increases the nonquantal release of ACh as well. Malomouzh and colleagues (2011) established that the inhibitory effect of ATP on the nonquantal release of ACh occurs through the phospholipase C via metabotropic P2Y purinergic receptors. Adenosine is a neurotransmitter that synchronises the release of quantal release of ACh. It is a product of the breakdown of adenosine 5’ triphosphate and acts at the inhibitory adenosine A1 and facilitatory A2a receptors. Activation of A1 receptors reduces the number of ACh molecules released in each quantum. Increasing intracellular Ca2 + in the nerve terminal activates the exocytotic process that is mediated by A2a receptors. The A2a receptors also contribute to the facilitatory effect of CGRP on the release of ACh. In addition, modulation of a quantal ACh release occurs through secondary messenger systems involving protein kinase A and C (PKA and PKC). The potential role of CGRP in myofascial pain and other pain conditions such as migraines cannot be underestimated. CGRP is found in higher concentrations in the immediate environment of active TrP (Shah et al., 2008). It is a potent microvascular vasodilator involved in wound healing, prevention of ischemia, and several autonomic and immune functions (Smillie & Brain, 2011). CGRP and its receptors are widely expressed in the central and peripheral nervous system. For example, CGRP type I is produced in the cell body of motor neurons in the ventral horn of the spinal cord and is excreted via an axoplasmatic transport mechanism. CGRP is also released from the trigeminal ganglion and from trigeminal nerves within the dura and, as such, contributes to peripheral sensitisation (Durham & Vause, 2010). It stimulates the phosphorylation of ACh receptors, which prolongs their sensitivity to ACh (Hodges-Savola & Fernandez, 1995). In addition, CGRP promotes the release of ACh and inhibits AChE. Interestingly, myofascial tension, as seen with TrPs, may also stimulate an excessive release of ACh, which suggests the presence of a self-sustaining vicious cycle (Chen & Grinnell, 1997; Grinnel et al., 2003). Experimental research of rodents demonstrated that excessive ACh in the synaptic cleft leads to morphological changes resembling TrP contractures (Mense et al., 2003). Consuming excessive amounts of coffee in combination with alcohol triggers a similar response pattern, which has been attributed to the ability of caffeine to release Ca2 + from the sarcoplasmic reticulum (Oba et al., 1997a, 1997b; Shabala et al., 2008). Many human, rabbit, and equine studies of TrPs have confirmed the presence of spontaneous electrical activity of continuous low amplitude referred to as endplate noise (EPN) and intermittent large amplitude spikes referred to as endplate spikes (EPS) (Bron and Dommerholt, 2012). The prevalence of EPN and EPS is correlated with the degree of irritability and pain (Kuan et al., 2007b). Of interest is a recent study of dry needling at myofascial trigger spots in model rats, which showed increased levels of ACh and nAChr at TrP locations, but not in controls without TrPs or in non-TrP sites (Liu et al., 2017). Dry needling caused a significant decrease in ACh, nAChRs, EPN, and EPS. EPN and EPS are hypothesised to be the result of an excess release of ACh at the motor endplate. Failure of the motor endplate is likely an intrinsic dysfunction of the endplate itself, as the degree of endplate noise does not change when the motor nerve is transected (Hong and Yu, 1998). Manual strumming or needling of a TrP usually result in a so-called local twitch response (LTR), which is a sudden contraction of muscle fibres in a taut band (Hong & Simons, 1998). LTRs can be observed visually, can be recorded electromyographically, or can be visualised with diagnostic ultrasound (Gerwin & Duranleau, 1997; Rha et al., 2011). The number of LTRs may be related to the irritability of the muscle TrP (Hong et al., 1997), although some muscles such as the triceps brachii commonly feature many LTRs. The irritability appears to be correlated with the degree of sensitisation of muscle nociceptors by bradykinin, serotonin, and prostaglandin, among others. Hong and Torigoe (1994) reported that LTRs could be elicited in an animal model by needling of hypersensitive trigger spots, which are the equivalent of TrPs in humans. LTRs were observed in only a few of the control sites. In addition, LTRs could not be elicited after transection of the innervating nerve. LTRs are spinal cord reflexes elicited by stimulating the sensitive site in the TrP region (Hong et al., 1995). Audette and colleagues (2004) reported that dry needling of active TrPs in the trapezius and levator scapulae muscles elicited bilateral LTRs in 61.5% of the muscles, whereas dry needling of latent TrPs resulted only in unilateral LTRs. Eliciting LTRs has been advocated for effective TrP dry needling (Hong, 1994). After LTR, Shah and colleagues (2005) observed an immediate drop in concentrations of several neurotransmitters, including CGRP and substance P, and several cytokines and interleukins in the extracellular fluid of the local TrP milieu. The concentrations did not quite reach the levels of normal muscle tissue. The reductions were observed during approximately 10 minutes before they appeared to slowly rise again. Due to the short observation times, it is not known whether the concentrations stabilised or increased again over time. Recently several authors have questioned whether eliciting LTRs is necessary or even desirable (Koppenhaver et al., 2017; Perreault et al., 2017), which will be addressed in more detail in Chapter 2. Muscle pain follows noxious stimuli, which activate specific peripheral nociceptors. If nociceptive impulses exceed the threshold of various nociceptors, they are transmitted through second order neurons in the dorsal horn, through the spinal cord, and to primary and secondary somatosensory areas in the brain, including the amygdala, anterior cingulated gyrus, and the primary sensory cortex. Locally, activation of receptors leads to the release of neuropeptides, which also causes vasodilatation and increase the permeability of the microvasculature (Snijdelaar et al., 2000; Ambalavanar et al., 2006). When neuropeptides are released in sufficient quantity, they trigger the release of histamine from mast cells, BK from kallidin, 5-HT from platelets, and PGs from endothelial cells (Massaad et al., 2004); this release leads to a vicious cycle as these chemicals also activate peripheral nociceptive receptors and potentiate dorsal horn neuron sensitisation. As such, muscle nociceptors play an active role in muscle pain and in the maintenance of normal tissue homoeostasis by assessing the peripheral biochemical milieu and by mediating the vascular supply to peripheral tissue. The responsiveness of receptors is indeed a dynamic process and can change depending on the concentrations of the sensitising agents. As an example, under normal circumstances the BK receptor, knows as a B2 receptor, triggers only a temporary increase of intracellular calcium and does not play a significant role in sensitisation. When the BK concentration increases, a B1 receptor is synthesised that facilitates a long-lasting increase of intracellular calcium and stimulates the release of tumour necross factor and other interleukins, which in turn lead to increased concentrations of BK and peripheral sensitisation (Calixto et al., 2000; Marceau et al., 2002). There are many interactions between these chemicals making muscle pain a very complicated phenomenon. Babenko and colleagues (1999) found that the combination of BK and 5-HT induced higher sensitisation of nociceptors than each substance in isolation. Referred pain occurs at the dorsal horn level and is the result of activation of otherwise quiescent axonal connections between affective nerve fibres dorsal horn neurons, which are activated by mechanisms of central sensitisation (Mense & Gerwin, 2010). Referred pain is not unique to myofascial TrPs, but, nevertheless, it is highly characteristic of myofascial pain syndrome. Usually referred pain happens within seconds after mechanical stimulation of active TrPs, suggesting that the induction of neuroplastic changes related to referred pain is a rapid process. Kuan and colleagues (2007a) demonstrated that TrPs are more effective in inducing neuroplastic changes in the dorsal horn neurons than non-TrPs regions. The multiple contractures found in muscles of patients with myofascial pain can compress regional arteries, resulting in ischemia and hypoxia. Recent Doppler ultrasound studies confirmed a higher outflow resistance or vascular restriction at active TrPs and an increased vascular bed outside the immediate environment of TrPs, which is consistent with the measurement of decreased oxygen saturation levels within TrPs and increased levels outside the core of TrPs (Brückle et al., 1990; Ballyns et al., 2011). Hypoxia may trigger an immediate increased release of ACh at the motor endplate (Bukharaeva et al., 2005). Hypoxia also leads to a decrease of the local pH, which will activate transient receptor potential vanilloid (TRPV) receptors and acid sensing ion channels (ASIC), among many others, via hydrogen ions or protons. Because these channels are nociceptive, they initiate pain, hyperalgesia, and central sensitisation without inflammation or any damage or trauma to the muscle (Sluka et al., 2001, 2002, 2003, 2009; Deval et al., 2010). Research at the US National Institutes of Health has confirmed that the pH in the direct vicinity of active TrPs is well below 5 (Shah et al, 2005), which is sufficient to activate muscle nociceptors (Sahlin et al., 1976; Gautam et al., 2010). Different kinds of ASICs play specific roles (Walder et al., 2010), and it is not known which specific ASICs are activated in myofascial pain. It is likely that multiple types of ASICs are involved in the sensory aspects of TrPs (Dommerholt, 2011) such as the ASIC1a, which processes noxious stimuli, and the ASIC3, which is involved in inflammatory pain (Shah et al., 2005; Deval et al., 2010). A low pH down-regulates AChE at the neuromuscular junction and can trigger the release of several neurotransmitters and inflammatory mediators such as CGRP, substance P, BK, interleukins, ATP, 5-HT, PG, potassium, and protons; this would result in a decrease in the mechanical threshold and activation of peripheral nociceptive receptors. A sensitised muscle nociceptor has a lowered stimulation threshold into the innocuous range and will respond to harmless stimuli such as light pressure and muscle movement. When nociceptive input to the spinal cord is intense or occurs repeatedly, peripheral and central sensitisation mechanisms occur and spread of nociception at the spinal cord level results in referred pain (Hoheisel et al., 1993). As stated, muscle pain is associated with the activation of muscle nociceptors by a variety of endogenous substances, including several neuropeptides and inflammatory mediators, among others. In experimental research different substances are commonly used to elicit local and referred muscle pain (Babenko et al., 1999; Arendt-Nielsen et al., 2000; Arendt-Nielsen & Svensson, 2001; Graven-Nielsen, 2006). In fact, induced referred pain areas obtained in these experimental studies have confirmed the empirical referred pain patterns described by Travell and Simons (Travell & Rinzler, 1952; Simons et al., 1999). Peripheral sensitisation is described as a reduction in the pain threshold and an increase in responsiveness of the peripheral nociceptors. Scientific evidence has shown that pressure sensitivity is higher at TrPs than at control points (Hong et al., 1996), suggesting an increased nociceptive sensitivity at TrPs and peripheral sensitisation. The concentrations of BK, CGRP, substance P, tumour necrosis factor α (TNF-α), interleukins 1β, IL-6, and IL-8, 5-HT, and norepinephrine were significantly higher near active TrPs than near latent TrP or non-TrP points and in remote pain-free distant areas (Shah et al., 2005, 2008). These chemical mediators may partly be released from peripheral sensitised nociceptors that drive the pain, but also be released from the sustained muscle fibre contraction within the taut band (Gerwin, 2008). Interestingly, the concentrations of these biochemical substances in a pain-free area of the gastrocnemius muscle were also higher in individuals with active TrPs in the upper trapezius muscle compared with those with latent TrPs or non-TrPs (Shah et al., 2008). These studies confirm not only the presence of nociceptive pain hypersensitivity in active TrP, but also establish that TrPs are a focus of peripheral sensitisation. Substances associated with muscle pain and fatigue are apparently not limited to local areas of TrPs or a single anatomical locus. Li and colleagues (2009) reported nociceptive (hyperalgesia) and nonnociceptive (allodynia) hypersensitivity at TrPs, suggesting that TrPs sensitise both nociceptive and nonnociceptive nerve endings. Nevertheless, painful stimulation induced higher pain response than nonnoxious stimulation at TrPs (Li et al., 2009). Hsieh and colleagues (2012) confirmed that the concentrations of multiple chemicals, including β-endorphin, substance P, TNF-α, cyclooxygenase-2 (COX-2), hypoxia-inducible factor-1α (HIF-1α), inducible isoform of nitric oxide synthases (iNOS), and vascular endothelial growth factor (VEGF) were significantly higher. Of interest is that dry needling reduced these concentrations, but this was dosage dependent (Hsieh et al., 2012). Wang and colleagues (2010) reported that ischemic compression, which mainly blocked large-diameter myelinated muscle afferents, induced increased pressure pain and referred pain thresholds at the TrP, but not at non-TrP regions. After decompression, the pressure sensitivity returned to precompression levels. In other words, nonnociceptive large-diameter myelinated muscle afferents may be involved in the pathophysiology of TrP pain and hyperalgesia (Wang et al., 2010). As nonnociceptive afferents are involved in proprioception, excitation of the large-diameter myelinated afferents by TrPs may explain the presence of altered proprioception in some patients with chronic musculoskeletal pain. Meng and colleagues (2015) confirmed that myelinated afferents influence endplate noise and mechanical hyperalgesia in a rat model. Central sensitisation is an increase in the excitability of neurons within the central nervous system, characterised by allodynia and hyperalgesia. Hyperalgesia is an increased response to a stimulus that is normally painful. Allodynia and hyperalgesia are observed in patients with TrPs. In fact, emerging research suggests a physiological link between the clinical manifestations of TrPs, such as hyperalgesia and consistent referred pain, and the phenomenon of central sensitisation, although the causal relationships and mechanisms are still unclear. Additionally, Arendt-Nielsen and colleagues (2008) demonstrated that experimentally induced muscle pain is able to impair diffuse noxious inhibitory control mechanisms, supporting an important role of muscle tissues in chronic pain. Mense (1994) suggested that the presence of multiple TrPs in the same or different muscles or the presence of active TrPs for prolonged periods of time may sensitise spinal cord neurons and supraspinal structures by means of a continued peripheral nociceptive afferent barrage into the central nervous system. Both spatial and temporal summations are important in this pattern. Although the relationship between active TrPs and central sensitisation has been observed clinically for many years, neurophysiological studies have been conducted only during the last decade. Kuan and colleagues (2007a) reported that spinal cord connections of TrPs were more effective in inducing neuroplastic changes in the dorsal horn neurons than non-TrPs. In addition, motor neurons related to TrPs had smaller diameters than neurons of normal tissue. It appears that TrP may be connected to a greater number of small sensory or nociceptive neurons than non-TrP tissues (Kuan et al., 2007a). Mechanical stimulation of latent TrPs can induce central sensitisation in healthy subjects, suggesting that stimulation of latent TrPs can increase pressure hypersensitivity in extrasegmental tissues (Xu et al., 2010). Central sensitisation also increased the TrP pressure sensitivity in segmentally related muscles (Srbely et al., 2010a). Further, Fernández-Carnero and colleagues (2010) reported that central sensitisation related to TrPs in the infraspinatus increased the amplitude of electromyographical (EMG) activity of TrPs in the extensor carpi radialis brevis. Current evidence suggests that TrPs induce central sensitisation, but sensitisation mechanisms can also promote TrP activity (Fernández-de-las-Peñas & Dommerholt, 2014). It is, however, more likely that TrPs induce central sensitisation, as latent TrPs are present in healthy individuals without evidence of central sensitisation. Finally, active TrP pain is, at least partially, processed at supraspinal levels. Recent imaging data suggest that TrP hyperalgesia is processed in various brain areas as enhanced somatosensory activity involving the primary and secondary somatosensory cortex, inferior parietal, and mid-insula and limbic activity, involving the anterior insula. Suppressed right dorsal hippocampal activity is present in patients with TrPs in the upper trapezius muscle compared with healthy controls (Niddam et al., 2008; Niddam, 2009). Abnormal hippocampal hypoactivity suggests that dysfunctional stress responses play an important role in the generation and maintenance of hyperalgesia from TrPs (Niddam et al., 2008). Current data suggest that a TrP is more painful than normal tissue because of specific physiological changes as well as peripheral and central sensitisation, and not because of anatomical issues. A sensitised central nervous system may modulate referred muscle pain. Infusions with the N-methyl-D-aspartate (NMDA) antagonist ketamine in individuals with fibromyalgia reduced their referred pain areas (Graven-Nielsen et al., 2000). As noted previously, the appearance of new receptive fields is characteristic of muscle and fascia referred pain (Mense, 1994; Taguchi et al., 2008; Schilder et al., 2014). Because referred pain area is correlated with the intensity and duration of muscle pain (Graven-Nielsen et al., 1997b), muscle referred pain appears to be a central sensitisation phenomenon maintained by peripheral sensitisation input for example from active TrPs. Central sensitisation is a reversible process in patients with myofascial pain, although older animal studies suggest that central sensitisation would be an irreversible process (Sluka et al., 2001). Several clinical studies have demonstrated that sensitisation mechanisms related to TrPs may be reversible with proper management. TrP injections into neck muscles produced a rapid relief of palpable scalp or facial tenderness, which would constitute mechanical hyperalgesia and allodynia, and associated symptoms in migraine (Mellick & Mellick, 2003). Anaesthetic injections of active TrPs significantly decreased mechanical hyperalgesia, allodynia, and referred pain in patients suffering from migraine headaches (Giamberardino et al., 2007), fibromyalgia (Affaitati et al., 2011), and whiplash (Freeman et al., 2009). In addition, dry needling of primary TrPs inhibited the activity in satellite TrPs situated in their zone of referred pain (Hsieh et al., 2007). Dry needling of active TrPs has been shown to temporarily increase the mechanical pain threshold in local pain syndromes (Srbely et al., 2010b), suggesting a segmental antinociceptive effect of TrP therapy. The cause of the rapid decrease in local and referred pains with manual TrP therapy observed in clinical practice is not completely understood but may be, at least partially, the result of the mechanical input from the needle, which would cause local stretching of muscle fibres, elongation of fibroblasts, and microdamage to tissues (Domingo et al., 2013). The resolution of referred pain is related to the decrease in nociceptive input to the dorsal horn of the spinal cord, and interruption of the spread of pain through convergence and central sensitisation. Nevertheless, the reversal in referred pain is surprisingly fast and suggests that long-standing central sensitisation can be reversed instantaneously with proper treatment. This effect may be related to the up-regulation of the endocannabinoid or endorphin system as a result of myofascial manipulation and other soft tissue therapies (McPartland, 2008). Empirically, dry needling and TrP injections have a much quicker result than strict manual TrP release techniques, presumably due to increased specificity of the stimulus (Dommerholt & Gerwin, 2010). In spite of methodological limitations of many studies, there is ample evidence that manual techniques are effective (Hains et al., 2010a, 2010b; Hains & Hains, 2010; Bron et al., 2011b; Rickards, 2011) without any indication that one particular manual technique would be superior to another (Fernández-de-las-Peñas et al., 2005; Gemmell et al., 2008). Accumulated evidence indicates that referred pain is a reversible process of central nervous system neuroplasticity (Arendt-Nielsen et al., 2000), which is maintained by increased peripheral nociceptive input from active TrPs. It is conceivable that the degree of central sensitisation may influence whether a patient will eventually be diagnosed with myofascial pain, fibromyalgia, or neuropathic pain. Multiple factors can influence the degree of sensitisation including descending inhibitory mechanisms, sympathetic activity, or neuropathic activation. In clinical practice it is commonly seen that patients with less central sensitisation require a fewer number of treatments. There is a growing interest in the association between TrPs and the sympathetic nervous system. Rabbit (Chen et al., 1998) and human studies (McNulty et al., 1994; Chung et al., 2004) showed that an increased sympathetic efferent discharge increased the frequency and the amplitude of EMG activity of muscle TrPs, whereas sympathetic blockers decreased the frequency and amplitude of EMG activity. Others reported that sympathetic blockers decreased TrP and tender point pain sensitivity (Bengtsson & Bengtsson, 1988; Martinez-Lavin, 2004), which is consistent with the observed increased concentrations of norepinephrine at active TrPs (Shah et al., 2005). Ge and colleagues (2006) reported that sympathetic facilitation induced a decrease in the pressure pain thresholds and pressure threshold for eliciting referred pain and an increase in local and referred pain intensities, suggesting a sympathetic-sensory interaction at TrPs. Zhang and colleagues (2009) found an attenuated skin blood flow response after painful stimulation of latent TrPs compared with non-TrPs, which may be secondary to increased sympathetic vasoconstriction activity at latent TrPs. TrP sensitivity appears to be maintained by sympathetic hyperactivity, although, once again, the mechanisms of this interaction are not completely understood. Increased sympathetic activity at TrPs may enhance the release of norepinephrine and ATP, among others (Gerwin et al., 2004). Another possible mechanism suggests that the increased level of muscle sympathetic nerve activity may lead to a delayed resolution of inflammatory substances and change the local chemical milieu at TrPs (Macefield & Wallin, 1995). As was discussed previously, Gerwin and colleagues (2004) suggested that the presence of α and β adrenergic receptors at the endplate could provide a possible mechanism for autonomic interaction (Maekawa et al., 2002), although this has not been confirmed in humans. The activation of a TrP may result from a variety of factors such as repetitive muscle overuse, acute or sustained overload, psychological stress, or other key or primary TrPs. Particular attention has been paid to injured or overloaded muscle fibres after eccentric and intense concentric contractions in the pathogenesis of TrPs (Gerwin et al., 2004). Hong (1996) hypothesised that each TrP contains a sensitive locus, described as a site from which a LTR can be elicited when the TrP is mechanically stimulated, and an active locus, described as an area from which spontaneous electrical activity (SEA) is recorded. In this model, the sensitive locus contains nociceptors and constitutes the sensory component, whereas the active locus consists of dysfunctional motor endplates, which would be the motor component (Simons et al., 1995; Simons, 1996; Hong & Simons, 1998). To date, the presence of these loci has not been confirmed. Muscle trauma, repetitive low-intensity muscle overload, or intense muscle contractions may create a vicious cycle of events, wherein damage to the sarcoplasmic reticulum or the cell membrane leads to an increase of the calcium concentration, a shortening of the actin and myosin filaments, a shortage of ATP, and an impaired calcium pump (Simons et al., 1999; Gerwin et al., 2004). This vicious cycle leads to the development of the so-called ‘energy crisis hypothesis‘ (Simons & Travell, 1981), which over time evolved into the integrated trigger point hypothesis, based on subsequent scientific research (Simons, 2004). The integrated hypothesis is the most accepted theoretical concept, although other models have been proposed (Dommerholt & Franssen, 2011). The integrated hypothesis is a work in progress and continues to be modified and updated as new scientific evidence emerges (Gerwin et al., 2004; McPartland & Simons, 2006; Jafri, 2014). The integrated hypothesis proposes that abnormal depolarisation of the postjunctional membrane of motor endplates causes a localised hypoxic energy crisis associated with sensory and autonomic reflex arcs that are sustained by complex sensitisation mechanisms (McPartland & Simons, 2006). The first EMG study of TrPs, conducted by Hubbard and Berkoff (1993), reported the presence of spontaneous EMG activity in a TrP of the upper trapezius muscle. The authors described two components of this spontaneous EMG activity: a low amplitude constant background activity of 50 μV and an intermittent higher amplitude spikelike of 100 to 700 μV. Others confirmed the constant background activity of 10 to 50 μV and, occasionally, 80 μV in animal TrPs (Simons et al., 1995; Chen et al., 1998; Macgregor et al., 2006) and in human TrPs (Simons, 2001; Couppé et al., 2001; Simons et al., 2002). The origin of this SEA is still controversial; however, clear evidence supports that SEA originates from motor endplate potentials (EPP). Simons concluded that the SEA is the same as endplate noise (EPN) (Simons, 2001; Simons et al., 2002). EPN is more prevalent in active TrPs than in latent TrPs (Mense & Gerwin, 2010). EPN seems to reflect a local depolarisation of the muscle fibres induced by a significantly increased and abnormal spontaneous release of ACh (Ge et al., 2011). Kuan and colleagues (2002), in an animal model, showed that SEA can be decreased by botulinum toxin, which inhibits the release of ACh, CGRP, and other chemicals at the neuromuscular junction. Additionally, analysis of the motor behaviours of a TrP shows that the intramuscular EMG activity at TrPs exhibits similar motor behaviours to the surface EMG activity over a TrP, which supports that the origin of the electrical activity is derived from extrafusal motor endplates and not from intrafusal muscle spindles (Ge et al., 2011). An interesting study found higher pain intensities and pain features similar to TrPs when noxious stimuli were applied to motor endplate areas compared with silent muscle sites (Qerama et al, 2004). Kuan and colleagues (2007b) reported a high correlation between the irritability, pain intensity, and pressure pain thresholds and the prevalence of EPN loci in a TrP region of the upper trapezius muscle. Lower pressure pain thresholds were associated with greater SEA. From a clinical perspective, several studies showed that treatment of TrPs can eliminate or significantly reduce EPN (Kuan et al., 2002; Gerwin et al., 2004; Qerama et al., 2006; Chen, Hong et al., 2008; Chou et al., 2009). Findings from these studies support that TrPs are associated with dysfunctional motor endplates (Simons et al., 2002). It should be noted that motor endplates are distributed throughout the entire muscle and not just in the muscle belly as frequently is assumed (Edström & Kugelberg, 1968). In studies of cats and rats, motor endplates were identified in 75% of the soleus muscle (Bodine-Fowler et al., 1990; Monti et al., 2001). In the anterior tibialis muscle of a cat, motor endplates were located in 56% to 62% of the muscle (Monti et al., 2001). Regarding the motor component of the TrPs, the intramuscular and surface EMG activity recorded from a TrP showed that the SEA is similar to a muscle cramp potential and that the increase in local muscle pain intensity is positively associated with the duration and amplitude of muscle cramps (Ge et al., 2008). Localised muscle cramps may induce intramuscular hypoxia, increased concentrations of algogenic mediators, direct mechanical stimulation of nociceptors, and, subsequently, the experience of pain (Simons & Mense, 1998). Therefore it seems that TrP pain and tenderness are closely associated with sustained focal ischemia and muscle cramps within muscle taut bands (Ge et al., 2011). Although current evidence supports that dysfunctional motor endplates are clearly associated with TrPs, recent evidence suggests that muscle spindles may also be involved in this complex process. Ge and colleagues (2009) found that intramuscular TrP electrical stimulation can evoke H-reflexes and that greater H-reflex amplitudes and lower H-reflex thresholds exist at TrPs compared with non-TrPs. The lower reflex threshold and higher reflex amplitude at TrPs could be related to a greater density or excitability of muscle spindle afferents (Ge et al., 2009). Nevertheless, the mechanisms underlying increased sensitivity of muscle spindle afferents at TrPs are still unclear. The increased chemical mediators in the TrPs (Shah et al., 2005) may contribute to an increased static fusimotor drive to muscle spindles or to increased muscle spindle sensitivity (Thunberg et al., 2002). Recently Jafri (2015) expanded the integrated hypothesis by incorporating the role of reactive oxygen species (ROS). The integrated hypothesis postulated that when Ca2 + is not removed from the cytosol, the actin-myosin crossbridge would remain. Removing Ca2 by reuptake into the sarcoplasmic reticulum is an energy demanding process that occurs via the Na+/K+-ATPase (sarcoendoplasmic reticulum ATPase [SERCA]) system. In Jafri’s point of view, the role of Ca2 + has been undervalued. He summarised that increased muscle activity, as seen in muscle overload, generates ROS, especially superoxide. Normally, ROS are converted by superoxide dismutase (SOD), which converts superoxide to hydrogen peroxide (H2O2). Eventually H2O2 is broken down to oxygen and water by glutathione peroxidase or catalase. Under stressful or pathological conditions, the enzyme xanthine oxidase and phospholipase A2 dependent processes have been shown to also produce ROS. During repetitive contractions, nicotinamide adenine dinucleotide phosphate oxidase 2 (NOX2), located within the sarcoplasmic reticulum, the sarcolemma, and the transverse tubules, is the major source of superoxide ROS. Jafri hypothesised that mechanical stress can trigger an excessive release of Ca2 + in muscles through so-called X-ROS signaling. Mechanical deformation of the microtubule network can activate NOX2, which would produce ROS. The ROS oxidises ryanodine receptors leading to increases in Ca2 + release from the sarcoplasmic reticulum. The Ca2 + mobilisation resulting from mechanical stretch through this pathway is referred to as X-ROS signaling. In skeletal muscles, X-ROS sensitises Ca2 +—permeable sarcolemmal transient receptor potential or TRP channels, which may be a source of nociceptive input and inflammatory pain. Activating the transient receptor potential vanilloid 1 (TRPV1) leads to a quick increase in intracellular Ca2 + concentrations. Jafri suggested that myofascial pain is likely due to a combined activation of several ligand-gated ion channels, including the TRPV1 receptor, other acid sensing ion channels (ASIC3), BK and purinergic receptors, among others. Gerwin (2017) postulated that dysfunctional ATP-sensitive sodium channels (KATP receptor channel) may provide further answers to the pathophysiology of TrPs. When ATP concentrations are at normal levels, the KATP receptor channel is closed. When ATP levels drop, for example with hypoxia, the KATP receptor channel opens and inhibits the influx of Ca2 + from the sarcoplasmic reticulum and the endoplasmic reticulum into the cytosol and prevents the formation of ROS. Dysfunctional KATP receptor channels may trigger focal regions of supercontracted muscle fibres, which suggest that excessive ACh concentrations may not be the only mechanism leading to intense sarcomere contractures. Although the integrated trigger point hypothesis is the most prominent and most accepted model, other hypothetical TrP models have been developed. Recently Hocking (2010) postulated the central modulation hypothesis and suggested that plateau potentials are critical in the understanding of the aetiology of TrPs. According to Hocking, cell membranes may continue to trigger action potentials without synaptic excitation as a result of plateau potentials (Hocking, 2010). In other words, a sustained α-motoneuron plateau depolarisation would lead to the formation of TrPs. Hocking identified two underlying central nervous system mechanisms. So-called antecedent TrPs are thought to be the result of central sensitisation of C-fibre nociceptive withdrawal reflexes, visceromotor reflexes, or nociceptive jaw-opening reflexes; they occur in withdrawal reflex agonist muscles. Consequent TrPs would be due to compensatory reticulospinal or reticulotrigeminal motor facilitation; they occur in withdrawal reflex antagonist muscles. A critical difference with the integrated TrP hypothesis is that, in the central modulation model, myofascial pain is not a disorder of the motor endplate, but a nociception-induced central nervous system disorder leading to centrally maintained α-motoneuron plateau depolarisations (Hocking, 2010). There are several aspects of the integrated TrtP hypothesis that are also part of the central modulation hypothesis such as the presence of the energy crisis and low-amplitude motor endplate potentials. When Hocking presented his hypothesis initially, he also suggested several research projects to test the hypothesis. Further research is indeed needed to test this interesting hypothesis. Srbely (2010) developed the neurogenic hypothesis, which is based primarily on his and his associates’ research (Srbely & Dickey, 2007; Srbely et al., 2008, 2010a, 2010b). According to the neurogenic hypothesis, TrPs are neurogenic manifestations of primary pathologies in the same neurological segment. Srbely (2010) suggests that central sensitisation is the underlying cause of myofascial pain syndrome. The notion that the inactivation of TrPs can reverse central sensitisation is interpreted as evidence of the neurogenic hypothesis. Other than Srbely’s own studies, there is no other research to confirm or dispute the neurogenic hypothesis. Partanen and colleagues (2010) developed the neurophysiologic hypothesis, which maintains that the SEA is not recorded from motor endplates but from intrafusal muscle spindle fibres. According to this hypothesis, taut bands are caused by inflammation of muscle spindles and sensitisation of group III and IV afferents, which in turn leads to activation of the gamma and beta efferent systems (Partanen, 1999; Partanen et al., 2010). As was mentioned already, muscle spindles may be involved in the TrP aetiology (Ge et al., 2009), but there is no convincing evidence that endplate noise would originate in intrafusal fibres (Wiederholt, 1970). There is no research to date to confirm or dispute the neurophysiological hypothesis. Gunn (1997a, 1997b) developed the radiculopathy hypothesis, which is discussed in detail in Chapter 15. Quintner and colleagues (2015) suggested neuritis as a possible cause without providing any experimental support.
Basic Concepts of Myofascial Trigger Points
Myofascial trigger point overview
Neurophysiological basis of muscle referred pain
Clinical Characteristics of Muscle Referred Pain
Mechanisms and Neurophysiological Models of Referred Pain
Convergent-projection theory
Convergence-facilitation theory
Axon-reflex theory
Thalamic-convergence theory
Central hyperexcitability theory
Neurophysiological aspects of myofascial trigger points
The Nature of Trigger Points
Taut bands
The motor endplate
Local twitch response
Muscle pain
Sensitisation Mechanisms of Trigger Points
Trigger point as a focus of peripheral sensitisation
Trigger point nociception induces central sensitisation
Muscle referred pain is a process of reversible central sensitisation
Sympathetic facilitation of local and referred muscle pain
Pathophysiology of Trigger Points: The Integrated Hypothesis
Other Hypothetical Models
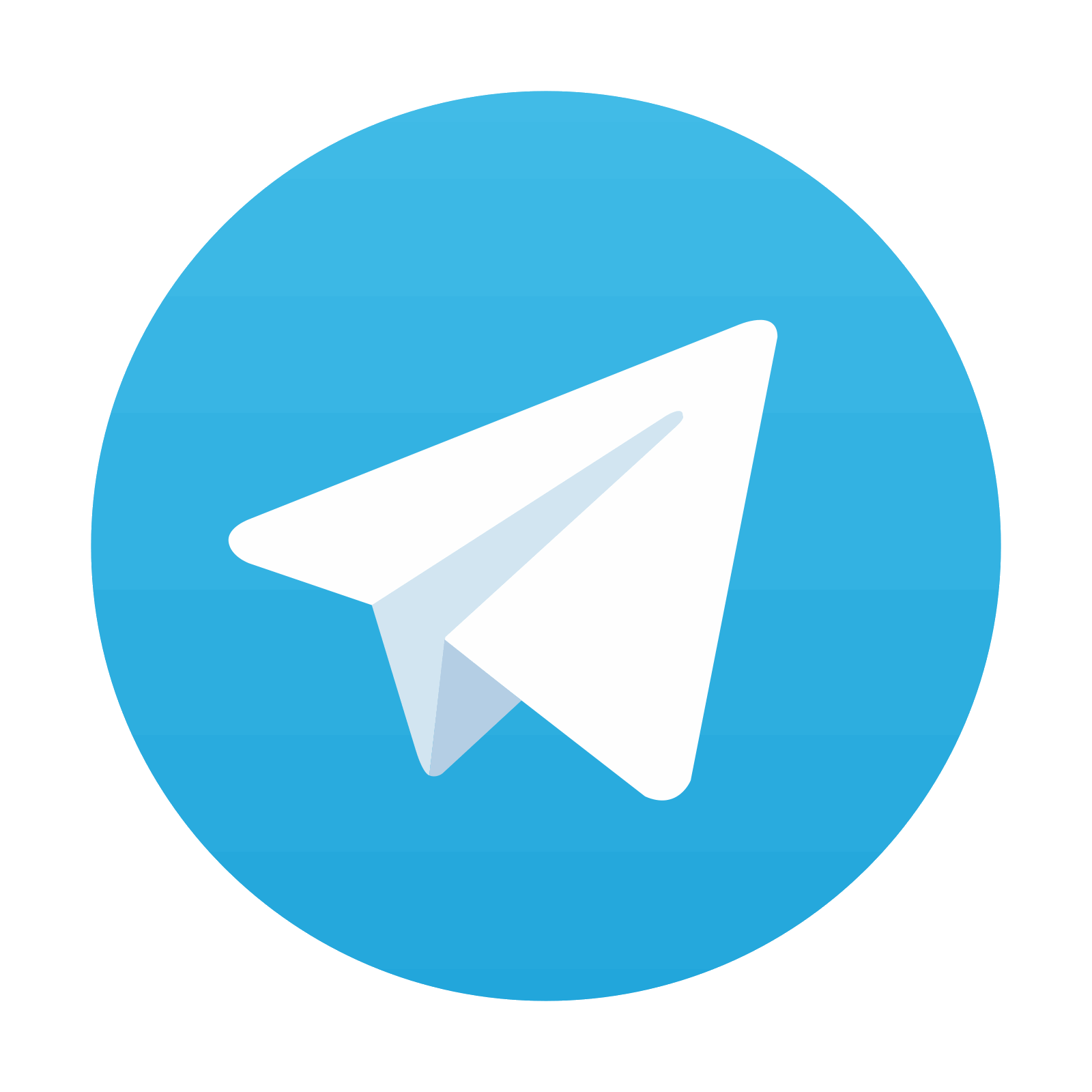
Stay updated, free articles. Join our Telegram channel
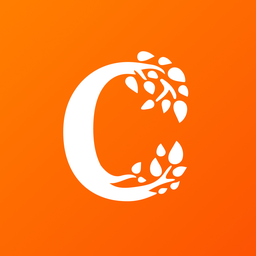
Full access? Get Clinical Tree
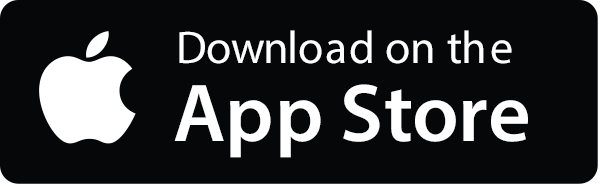
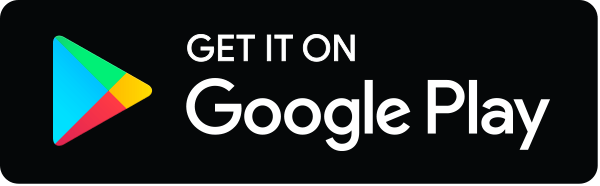