Lumbar disc , sagittal section; early degeneration, dehydrated and unstructured nucleus pulposus

Sagittal sections of lumbar spines in the newborn (a), young adult (b), and older adult (c)

Sagittal sections displaying various stages of degeneration in the same subject: L2-L3 normal disc (a), Medial fissure in L3-L4 disc (b), Posterior fissure in L4-L5 disc (c), and Loss of disc height in L5-S1 disc (d)
During aging, both the nucleus pulposus and annulus fibrosus become more fibrous and rich in collagen so that distinguishing them becomes less obvious in the elderly. Calcifications are formed preferentially at the level of annulus fibrosus but sometimes also affect the nucleus pulposus. Neo-angiogenesis recruiting pro-inflammatory cells was shown by Roberts et al. [4]. It occurs through damaged vertebral endplates and discal tears and consequently accelerates intervertebral disc ossification.

Cracks in the nucleus and annulus appear, hence causing disc rupture and nucleus ejection responsible for a herniated disc. The latter can therefore be considered as skipping a step in the aging process.

Disc and vertebral endplate modifications (a) Axial section of an intervertebral disk in a symptomatic subject: concentric annulus and laminate, eccentric nucleus pulposus. (b) Vertebral endplate showing a porous bone surface with annular ossification. (c) Changes due to aging: major circular osteophytes of the vertebral endplate giving a very irregular aspect
Type I combines a T1 hyposignal with T2 hyperintensity, which demonstrate hypervascularization and edematous inflammatory reactions adjacent to the bone marrow.
Type II comprises both T1 and T2 hyperintensity demonstrating a fatty involution of the bone marrow.
Type III, which is rarer, corresponds to fibrosis with poor vascularization and marked hyperostosis, equivalent to osteocondensation (Figs. 6, 7 and 8).



The last stage of disc degeneration represents disc fusion following hypomobility due to disc space narrowing and disc fibrosis, then anterior, posterior, and lateral osteophytosis.
Aggravating Factors
Aggravating or accelerating factors of such aging phenomena are mechanical, vascular, genetic, and most likely combined.
Mechanical Factors
Load repetition both in intensity and rhythm as well as vibrations leads to cartilaginous endplate calcification. Rotational movements expose to annular tears and aggravate the previously described fissuration phenomenon. According to Stokes and Iatridis [15], underuse may also display detrimental effects by decreasing cellular stimulation and altering nutrient transport. Disc overuse may also lead to degeneration. Examples include sudden shifts between mobile and stiff segments as seen in lumbosacral hinge anomalies (Bertolotti’s syndrome) or at surgical fusion extremities (junctional syndromes).
Experimental studies have permitted a better understanding of the effect of mechanical stresses on the intervertebral disc and its components.
Hutton et al. [16] showed the production increase of type 1 collagen in nucleus pulposus under the effect of compressive stresses. At the same time, they reported a decrease in type 2 collagen and proteoglycans production.
Handa et al. [17] reported from disc samples a decrease in proteoglycan production under high hydrostatic pressure (10 MPa), while synthesis was increased under low pressure (1 MPa).
Mechanical stresses that are directly applied to cells can modulate matrix protein synthesis [18]. The observed effects are likely to be dependent on the type of stress, its intensity, frequency, and duration.
Inflammatory Factors
The major role of inflammation was well-described by Rannou et al. [18]: certain metalloproteinases, cytokines, and growth factors are involved in disc degeneration with direct correlation between mechanical constraints and metalloproteinase activity. The production origin of these different factors is still debated: disc cells, neovascularization, or inflammatory granuloma.
Vascular Factors
Hypovascularization , especially microvascular disease, is also a factor of accelerated degeneration according to Kauppila et al. [19]. According to Urban et al. [5], smoking and vibrations also interfere by a vascular mechanism.
To date, studies reporting clinical and MRI observations of asymptomatic cohorts concerning the aging of the intervertebral disc mainly use disc-based classifications (derived from the Pfirmann classification [13]) without taking account of the endplate inflammatory reaction. Indeed, Modic types, often attributed to pathological mechanisms, are less studied in the context of asymptomatic subjects with aging spines.

Natural history of lumbar disc degeneration on MRI: loss of disc height and dehydration of lower discs, L5-S1 disc being affected first

Natural history of cervical discs on plain X-ray: initial loss of disc height in the lower cervical spine and hypermobility of higher discs
Zhang et al. [20] proposed, in a pilot study, the use of diffusion MRI to detect early disc behavioral abnormalities due to aging on a series of asymptomatic subjects at the molecular scale and in the absence of any morphological abnormality on T2 sequences. They suggested that the diffusion coefficient could decrease in degenerated discs and that it was correlated with the disc water content and the integrity degree of the disc matrix. It could also further specify the direction of the possible disc injury.
A meta-analysis published in 2014 by Brinjikji et al. [21] collected data on 3310 asymptomatic subjects with radiographic data (CT or MRI). Signs of disc degeneration were found as early as 20 years old (37% of asymptomatic subjects of that age group) and in 96% of subjects over 80 years old. A disc signal intensity decrease on T2-weighted images was present in more than half of the subjects over 40 years and 86% of the subjects over 60 years old. The disc height loss was correlated with age but to a lesser extent; however, 1% per year prevalence increase was noted. In contrast, the prevalence of disc protrusions and annular fissures did not seem to increase with age.
Pollintine et al. [22] highlighted an innovative biomechanical hypothesis regarding the absorption of compressive forces on the spine of elder patients. His cadaveric study on thoracolumbar pieces showed that aging caused a deformity of both vertebral bodies and intervertebral discs under compressive loads. Both structures displayed a substantial “creep.” The lower the disc pressures were, the more deformed the vertebrae were when placed under mechanical load.
Videman et al. [23] showed the natural history of lumbar intervertebral disc MRI morphology over 15 years on a large series of asymptomatic Finnish twins. The authors report a decrease in disc height averaging of 3.4% at 5 years and 8–11% at 15 years on MRIs performed during follow-up. An original aspect of that work consisted of showing a compensation by a vertebral height increase on incriminated levels of 3.1% (0.8 mm) in the higher lumbar spine and 4.7% (1.1 mm) in the lower lumbar spine. These preliminary results suggest a height increase of vertebral endplates compensating for multilevel disc height loss and thereby support Pollintine’s hypothesis .
Specific Features in the Cervical Spine
Disc degeneration plays a major role in the aging of the cervical spine. Okada et al. [24] showed a significant cervical spine morphological aging on MRI after a 10-year follow-up from a series of 223 asymptomatic subjects aged 39 ± 15 years. 34% of patients that were followed developed clinical symptoms including neck pain, shoulder stiffness, or subjective sensory disorders of their lower limbs; the onset of these symptoms was correlated disc degeneration visualized on MRI.
The same authors [25] analyzed the relationships between sagittal balance and disc degeneration in the cervical spine on a large cohort of asymptomatic subjects at a 10-year follow-up. There was no correlation between clinical symptoms and cervical spine sagittal balance. In their cohort, loss of disc signal intensity, posterior disc protrusions, and disc space narrowing were found in 64.6%, 65.5%, and 28.3%, respectively, of subjects at a 10-year follow-up. Subjects over 40 years old without cervical lordosis were the most likely to develop posterior disc protrusions.
Lumbar and Cervical Tandem Lesions
Okada et al. [25] demonstrated a higher prevalence of disc degeneration in the upper cervical spine in patients who already had lumbar disc herniations compared with asymptomatic controls.


Tandem lesions with cervical spinal stenosis (a, b, and c) with lumbar spinal stenosis (d and e)
Few studies were published regarding the aging of thoracic intervertebral discs. Of note, Matsumoto et al. [8] reported on MRI degeneration of thoracic intervertebral discs in 46.8% of subjects from an asymptomatic series of 94 subjects. Of these subjects, patients displayed a decrease in disc signal intensity on T2-weighted images, posterior disc protrusions, and compressions of the dural sac, respectively, 37.2%, 29.8%, and 30.9%. Only 4.3% presented disc space narrowing in the thoracic spine.
The thoracic spine, which has little mobility, therefore does not seem to be spared by these degeneration phenomena, despite greater mechanical constraints than in the cervical and lumbar spines, which are conversely lordosed and mobile.
The Posterior Arch
Facet Joints or Zygapophyseal Joints

Fissuring (cracking) of articular cartilage in the cervical spine, first stage of articular aging


Degeneration of the posterior articular muscles after Weishaupt et al. [26]: (a) normal articular space, (b) joint space narrowing, (c) geodes and osteophytes, (d) irregular cartilage and synovial cysts
Joint space narrowing is inexorable. Wang and Yang [27] has also shown progressive sagittalization of the lower lumbar facet joints, probably by increased constraints on the anterior and superior thirds of the superior facet of the lower vertebra, potentially leading to a degenerative spondylolisthesis (DSL).
Joint capsules can also be become hypertrophied along with a formation of posterior or anterior synovial cysts which may cause cauda equina with nerve root compression.
Cysts are associated with DSL in 60–89% of cases. A facet joint degeneration classification was proposed by Grogan et al. [28]. They distinguished four MRI stages of cartilaginous degeneration and four MRI stages of subchondral sclerosis.
Spinous Processes

Evolution toward kyphosis (b) with respect to the initial state (a) by disc narrowing (1), articular hypertrophy (2), hypertrophy of the spinous processes (c and d) with interspinous contact (3)
Ligaments
The ligament flavum has the highest elastin content in the human body and contains numerous proprioceptive fibers similarly to the multifidus muscle overlying its posterior aspect. As people age, its elastin content, and hence elasticity, decreases. Spinal ligaments can not only become hypertrophied and buckled due to disc height loss and facet joint overlap but they may also present with calcium pyrophosphate or hydroxyapatite deposits which are frequently found in lumbar spinal stenosis. Such deposits are not necessarily always associated with a history of chondrocalcinosis, hyperparathyroidism, or hemochromatosis.
The posterior longitudinal ligament and especially its midline fibers may become calcified, especially in the context of diffuse idiopathic skeletal hyperostosis (DISH).
Muscles
Sarcopenia is a geriatric syndrome characterized by loss of muscle mass and therefore decreased physical performance and strength. Spinal muscles are no exception to this. This phenomenon starts in the early thirties and accelerates after 50 years old: muscle mass decreases by 1–2% per year from this age while strength decreases by 1.5% per year. This corresponds to a loss of strength of 30% by decade after 60 years old. Both type I and type II muscle fibers are rarefied. Fiber atrophy is also observed and mostly affects fast type II fibers.
Stage 1: less than half the surface of affected muscles,
Stage 2: 50% fatty infiltration,
Stage 3: more than 50% of fat replacement (Fig. 15).

Interestingly, this fat replacement occurs from deep to superficial, the multifidus muscle being the first affected, and from the bottom up, that is from the lumbosacral junction to the thoracolumbar junction. Cruz et al. [31] demonstrated that there was a direct correlation between aging, loss of lumbar lordosis, and the importance of fatty infiltration of paraspinal muscles.

Histological abnormalities: (a) mitochondrial, (b) targetoid core, (c) ragged red fibers, (d) fibro-adipose

Modification of muscle fiber orientation on ultrasound with reduction of muscular fiber angle with age from (a) to (b) [33]
In summary, it should be remembered that aging is responsible for a reduction of muscle strength, especially in the lower limbs, of 1.5% per year after the age of 65. This is achieved by muscle fiber atrophy and type II fiber reduction with a power decrease of 3.5% per year. The same phenomena apply to the spine in conjunction with fatty infiltration of extensor muscles. The multifidus muscle, which is the most paramedian and closest to the lumbosacral junction structure, is first affected. Then, fatty replacement propagates laterally and cephalad. The psoas muscles remain unaffected for a long time.
Bone
Bone mineral density decreases with age due to hormonal and mechanical factors: type II or senile osteoporosis is distinct from type I postmenopausal osteoporosis or secondary osteoporosis.
in the sagittal plane, one drawn from the superior endplate toward the lamina and the inferior articular process, the other from the superior articular process to the inferior endplate (Fig. 18),
in the horizontal plane in a circular fashion around the spinal canal.

Bone trabeculae in the sagittal plane (a) and in the axial plane (b); the weak anterior triangle in bone trabeculae, the very anterior constraints explain the frequency of the anterior wedge-shaped vertebrae (c)
Bone strength depends on its density which directly depends on its thickness and number of bone trabeculae.

Evolution of the bony trabeculae: on a normal vertebra (a), on an osteoporotic vertebra (b); 1 = cartilage endplates, 2 = vertical trabeculae, 3 = horizontal trabeculae

Profiles of 100 elderly osteoporotic subjects according to Itoi [38]; types 4 and 5 are the most severe with compensation phenomena including pelvic retroversion and knee flexion

(a) The sagittal bipedal folding chain (BFC). (b) The frontal BFC. (c) The axial BFC
Aging and Neurological Control of Posture
With aging, apart from any pathology, deterioration of the neurological mechanisms responsible for postural stability occurs. The frequency and amplitude of postural oscillations increases along with a greater deviation from the gravity line. Visual, proprioceptive , and vestibular functions are altered. In the case of new sensory information, postural adjustments and the integration of sensory feedback loops are less effective. This leads to postural rigidification and alteration of the posture-movement coordination. Postural control is not only linked to spinal reflexes (muscle tone) and sensory information quality but also depends on cortical and subcortical systems involved in motor control and sensory integration. Aging requires a reorganization of cortical and spinal postural control. The aging subject will compensate via neurological and mechanical strategies.
Proprioception
Epicritic sensibility (touch and proprioception) provides important information for postural maintenance and necessary readjustments whether the subject is mobile or immobile.
Numerous factors are involved in proprioceptive aging [39]: decrease of intrafusal muscle fibers, muscle fiber modification, especially at the expense of type II fibers, reduction of receptors within muscles, tendons, joints, and skin, particularly in the foot arches, structural modification of peripheral nerve fibers (myelin reduction), especially for distal sensory fibers, with prolonged nerve conduction times; on examination, deep tendon reflexes are reduced or absent (the ankle jerk or Achilles reflex is absent in more than one-third of subjects over 70).
Plantar sensitivity decreases especially after the 7th decade. Receptors undergo morphological changes and their density decreases. Glabrous skin elasticity and nerve conduction decrease. The alteration of plantar sensitivity begins at the heel and secondarily affects the forefoot [40]. Visual conditions do not alter the distribution of plantar pressure. An adaptation strategy was proposed by moving plantar pressure to the forefoot [41]. The epicritic afferents from the foot arches constitute an important feedback for postural control for walking and even more so, orthostatism (orthostatic hypotension) [42].
Both plantar sensitivity and ankle dorsiflexors play a role in postural control. Elective vibratory stimulation of certain areas of the foot produces oriented postural responses with a displacement of the body to the opposite side. Plantar afferents could be a source of anticipation of the movements of the line of gravity during the oscillating phase of the step [40, 44].
The cervical spine: the receptor density within cervical muscles, especially in the suboccipital muscles, is largely higher than in most other muscles of the body.
With age, axial stiffness results in underuse of the oculocephalogyric system and loss of the head–trunk dissociation. Joint limitations and pain lead to a lack of proprioceptive information. As a result, abnormal information is sent to the vestibules [45]. A recent study showed that vibration exposure to the neck muscles reduces healthy subjects’ performance but improves that of the subjects with cervicalgia on postural control [46].
Despite its involvement in age-related postural disorders, the clinical evaluation of proprioception remains limited in the course of life. Several leads have nevertheless been proposed to improve postural control.
The alteration of type 2 fibers is partially reversible with exercise. Postural work (Tai Chi studies) enhances the proprioceptive capacities of the elderly. “Proprioceptive learning” must be done in conjunction with cognitive work [47]. Shoe adaptation is thought to increase proprioceptive feedback [41].
The maintenance of unipedal stance is a long-known indicator of fall risk. When walking, there is a step height and length decrease. Ankles are less solicited with age as balancing strategies reorganize around the hips.
Vision and Visual Motor Control
After 50 years old, there is a decrease in visual acuity, contrast sensitivity, darkness adaptation, accommodation, depth perception, and distance appreciation [48]. In orthostasis, the body oscillates on the ankles depending on the visual field displacement; the area of the postural oscillations increases by 30% standing with eyes closed [39]. Oscillations increase with age due to a poorer integration and interpretation of visuospatial information.
The Vestibule
The vestibulo-ocular reflex allows visual fixation during movements of the head. The vestibulospinal reflex stabilizes the head and posture in orthostasis by its facilitating action on the extensors of the head, trunk, and limbs.
The medial vestibulospinal tract, which projects bilaterally, facilitates the activity of cervical spine extensors. The lateral vestibulospinal tract, which is ipsilateral and fed by labyrinthine afferents, facilitates limb and trunk extensors activity.
However, in the absence of any pathology, as early as 50 years old, there is a decrease in sensory cells in the otolithic and semicircular systems, and in myelinated fibers from the vestibules. More than a third of individuals over 70 have bilateral vestibular disorders without any reported symptoms of instability [49].
Rotatory movements are integrated with more difficulty, due to an alteration of the lateral vestibulospinal tract which controls the thoracolumbar spine extensors, thus causing repercussions on the morphology of the lumbar spine.
Central Integration
All the structures of the central nervous system are involved in postural control: the tone of extensor muscles by vestibular, reticular, tectal, and olivary afferents; the perception of the vertical line (in the sagittal and coronal planes) by vestibular, thalamic, and cortical afferents; postural stabilization thanks to muscle synergy (basal ganglia) and muscular coordination (cerebellum).
Motor control.
The interaction between sensory information and its integration in the posterior association cortex. The perception of the vertical line is the result of a multisensory compromise. This is the basis of proposed rehabilitation programs: relationship between visual afferents and cervical proprioception [50]; postural exercises to improve vestibular control, with and without visual control, in individuals over 60 [51]. Sensory-motor reprogramming aims at teaching the elderly to use sensory input that was not used preferentially. The age-related quality deterioration of all sensory messages may lead to a real loss of afferent innervation (deafferenciation) [52].
Cognitive functions, especially by the prefrontal lobe, such as attentional ability, strategic behaviors, and memory processes.
All proprioceptive afferents are integrated centrally, either in the cerebellum or at the subcortical level, essentially in the minor hemisphere. Functional MRI studies have shown that putamen activation is correlated with proprioceptive capacity and decreases with age [53].
Sensorimotor postural control is hierarchized. Little attention is required, except in the elderly as they experience a decrease in information quality, information integration, and a reduction in automatic movement control. Thus, more attention is needed [54, 55]. Postural corrections are slower with a greater activation of the cortical and subcortical circuits required to maintain good performance [56]. The maintenance of the erect posture in the elderly subject negatively interacts with their ability to perform a second task [57, 58]. The deterioration of holokinetic motricity therefore imposes a greater cortical involvement. For example, subjects stop walking when talking.
Postural disturbances are common in Parkinson’s disease at an advanced clinical stage. They are associated with an orientation impairment to space, a trunk flexion tendency, and a decrease in postural correction reflexes [59].
Muscles work synergistically on the trunk and lower limbs and similarly on the neck and upper limbs to maintain posture. Muscle synergy corresponds to muscular activation patterns with reproducible and stable spatiotemporal characteristics, which activation threshold and latency are modified according to somatosensory information, thus allowing postural adjustments. Yet certain muscular synergies are thought to be adaptable and flexible [60].
Muscle synergies and their control could be quantified very early in the setting of the diagnosis of Parkinson’s disease even before postural problems translate into clinical symptoms.
Numerous tests have been and are being developed to assess the origin of postural disorders and to guide rehabilitation more specifically [61]. This allows the reorientation of rehabilitation in the case of sensory disorders, to compensate for a specific deficit (vestibulo-ocular or proprioception-vision relationships…). This minimizes the mechanical repercussions associated with postural disorders related to age or specific pathologies (static disorders, falls, lumbar spinal stenosis…).
Functional Alterations Associated with Aging
Postures and Dynamic Balances in Normal Adults
In all vertebrates , a polyarticular structure, foldable/unfoldable, made of articulated rigid segments was the solution selected by evolution to support the body and allows it to move in an unknown, irregular environment with multiple obstacles. Evolution favors flexibility at the expense of specialization, thus enabling a considerable number of tasks in the three spatial dimensions.
to provide a very strong and rigid frame thanks to the mechanical qualities of bone tissue and its adaptive remodeling capacities under the effect of repeated mechanical stresses,
to impose kinematic guidance to each segment and thus to achieve the same coordinate of the distal segment with a given approach vector, using several configurations of the proximal segments,
to amplify movements by summing angular motions, which leads to a remarkable acceleration of extremities.
Besides, this was the chosen solution to build humanoid robots.
However, despite the existence of numerous devices permitting automatic mechanical stops and cam effects (close-packed position), the precise control of the motions of each of the segments in the three spatial dimensions also requires the intervention of a large number of mono- or multi-articular muscles, mobilizing as well as stabilizing. Their programming and activation/coactivation must be constantly measured by nerve centers whose instructions are continuously running in perception/decision/action/feedback loops. Moreover, the main difficulty in anthropoid robotics lies precisely in fine task programming.
The stability of the body is not just an elementary mechanics problem that can be solved with some mathematical formulations. This is a much more complex problem involving a considerable number of regulations extrinsic to the musculoskeletal system. The kinematic study of this entire frame, that is the mechanical point-by-point calculation of the organization of each body element relative to each other at a given instant in the same inertial reference frame, is itself already terribly complex despite the development of new formulations of mechanical equations, modeling, and digital simulation.
Presently, it is illusory, even when limiting oneself to mechanics, to aim a complete representation of such a complex system as a whole at the risk of inducing inaccuracies and combinatorial explosion, i.e., chaotic results by unmanageable multiplication of data. For medical practice, rehabilitation and the analysis of the sporting gestures, the problem must be simplified, but at a level acceptable for the envisaged application.
To date, the only operational solution to facilitate data integration remains indirect. It consists of artificially moving information capture toward the projection of real events on each of the three anatomical reference planes: sagittal, frontal, and transverse. These planes do not have a physical existence. They are a theoretical construction intended to establish a simplified model of a mobile structure in an inertial reference frame which escapes our senses. These three planes have been favored because they are convenient for the teaching of anatomy (anatomical cross-sections) and also for the frontal and sagittal plane because they are easily accessible to physical examination and medical imaging. Thus, scoliosis specialists were the first to provide surgeons with geometrical parameters of normality formalized in these planes for sagittal and frontal balance. On these projections, the same foldable/unfoldable structures are observed. However each of them carries only partial information, yet different from that of other planes. It is from the study of all these data that one can extrapolate, as closely as possible, what can really happen, in particular thanks to 3D simulation and modeling.
Digital tools such as computed tomography, MRI, full-body weight-bearing EOS® 3D imaging, motion capture devices, and virtual reality are significantly enriching data capture. They will enable us, in the near future, to obtain a more and more precise idea of the exact nature of the structural and functional alterations which affect the vertebral system. Therefore, surgeons will be able to “keep a cool head” and predict side effects (or induced effects) of more and more demanding operative techniques. All the more so as in most cases, operative decisions are not urgent, as for the degenerative spinal deformity of the elderly.
However, the multiplicity of the parameters in question, both bony and non-skeletal, must increasingly encourage surgeons to adopt a holistic approach to patients before proposing therapeutic procedures that are in principle ideal and if possible definitive. We believe that only a systemic approach will advance knowledge in this area (cf. Chapter “Anatomy of the Spinal Meninges”).
Bipedal Folding Chains
We will refer to bipedal folding chains (BFC) as the projection, on each of the three anatomical reference planes, of the same skeletal device that regulates postural kinematics and movements of the erect man (Fig. 21). This denomination seems to us more direct and concise than “lumbo-pelvi-femoral complex” which seems too restrictive for us to describe all the osteoarticular components that contribute to body balance. Moreover, it neglects the essential role of the cervical spine and the distal segments of the lower limbs (knees, ankles, and feet). For example, characteristics of the human foot alone allow an anthropologist to isolate the Homo genus by implicitly evoking permanent bipedalism (Bennett 2009).
the entire spine from the foramen magnum to the sacrum,
the pelvic girdle,
and all the skeletal components of the lower limbs.
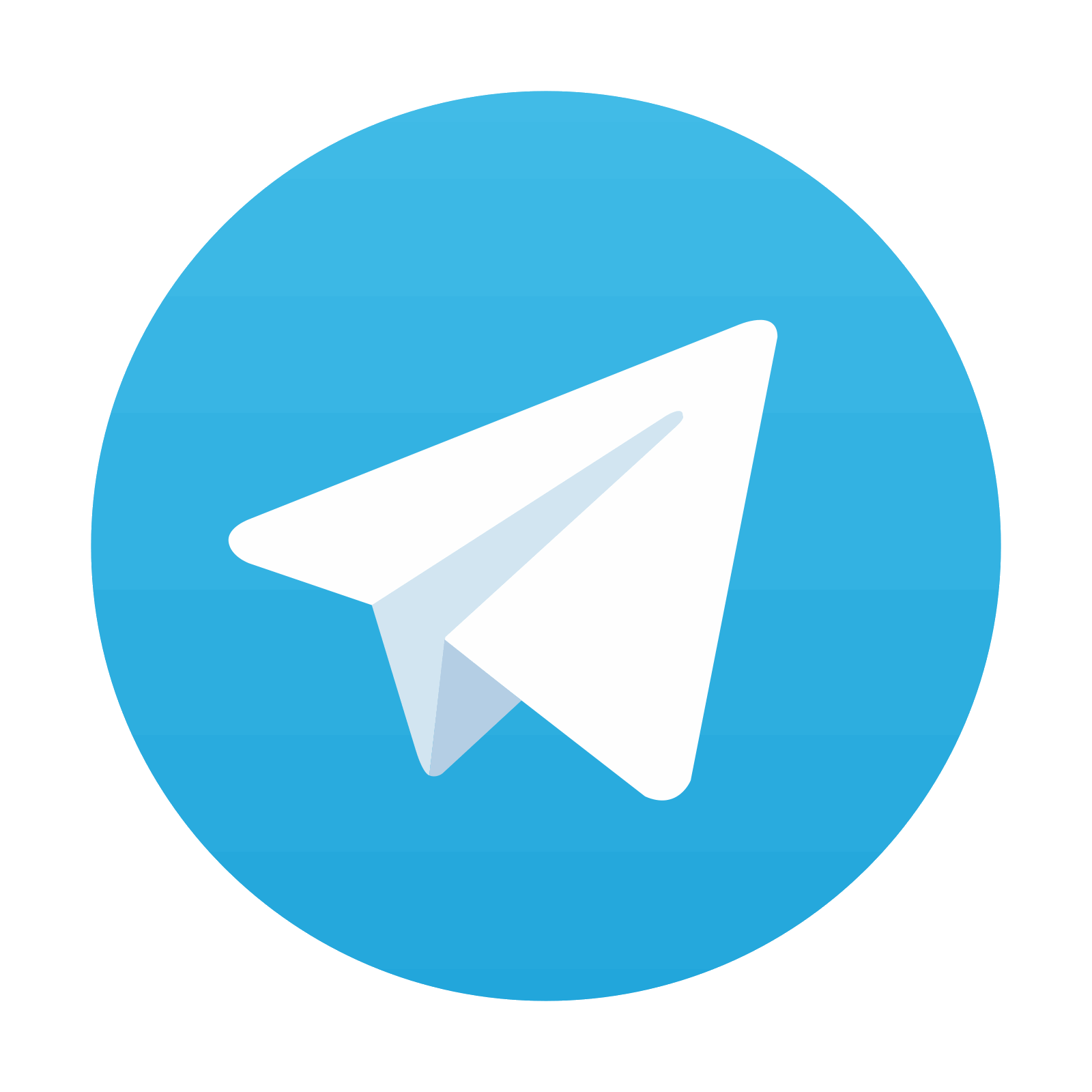
Stay updated, free articles. Join our Telegram channel
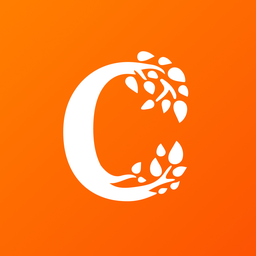
Full access? Get Clinical Tree
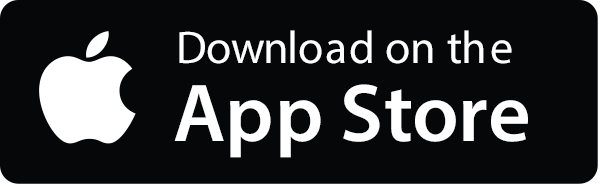
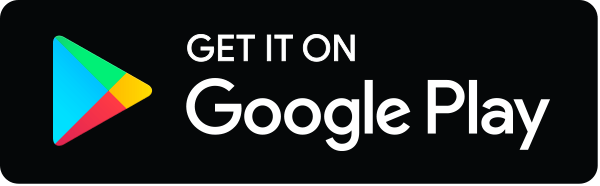