Advanced Rehabilitation for People with Microprocessor Knee Prostheses
Christopher Kevin Wong and Joan E. Edelstein
Learning Objectives
On completion of this chapter, the reader will be able to do the following:
2. Compare knee control function for a variety of microprocessor knee prostheses.
4. Apply Medicare K-level requirements when considering microprocessor knee prostheses for patients.
5. Explain the similarities and differences of microprocessor knee prostheses.
9. Describe the evidence to support use of microprocessor knee prostheses.
Historical development
Since Ambroise Parè’s sixteenth century articulated transfemoral prosthesis,1 surgeons, patients, and engineers have attempted to imitate the function of the human leg. In the United States, scientific prosthetics development began in 1945 with the establishment of the Prosthetic Appliance Service of the Veterans Administration and the research and development program of the National Academy of Sciences.2 Early versions of sophisticated knee units include the 1942 Filippi hydraulic stance control unit3 and the hydraulic swing and stance control knee unit patented by engineer Hans Mauch and radiologist Ulrich Henschke in 1949.4 The Veterans Administration approved the first hydraulic swing-phase control mechanism in 1962; the component linked a hydraulic knee unit to a single-axis ankle.5 This chapter discusses the unique features of microprocessor knee (MPK) prostheses that are increasingly used, and provides prosthetic and training solutions for persons with common gait deviations that can be reduced by using a MPK.
Research beginning in the 1970s led to the 1993 introduction by Blatchford (Basingstoke, England) of the first commercially available microprocessor-controlled prosthetic knee: the Endolite Intelligent Prosthesis. The Endolite Intelligent Prosthesis required a wired connection to program the variable swing-phase control. The Adaptive Prosthesis followed in 1998, allowing wireless programming and featuring an onboard processor that controlled adjustment of the hybrid pneumatic/hydraulic MPK; Endolite Intelligent Prosthesis (sixth generation) MPK is the Orion (Figure 27-1).6 Since introduction of the Endolite Intelligent Prosthesis, at least six other companies have joined the marketplace in offering MPK prostheses. Otto Bock (Duderstadt, Germany) initiated the hydraulic C-Leg MPK in 1997.2,7–10 Other manufacturers presented comparable units. Össur (Reykjavik, Iceland) launched the Rheo Knee in 2006 and the Power Knee in 2009.11 Freedom Innovations (Irvine, CA) recently introduced the Plié MPK unit.12 The Nabtesco Corporation of Japan also offers MPK units through the Swedish distributor, Centri AB.13
Overview of nonmicroprocessor knee prostheses
After amputation that includes the knee joint, people face significantly more difficulty in mobility tasks than those whose knees remain intact. Without the knee and the muscles that control it, the prosthesis user must control knee flexion in new ways to avoid falling. The simplest way to remain stable is to use a mechanically locked knee unit. Some older first-time prosthesis users prefer the security of a locked knee to one that is unlocked.14 If the knee is not locked, knee stability can be maintained simply through alignment of the joint axes combined with significant residual limb gluteal muscle power. However, many prosthesis users who wish to walk in the community with additional stability benefit from more sophisticated non-MPK units.
Weight-activated friction brake knees are non-MPK units that control knee flexion upon initial loading and through most of stance phase. Weight bearing on the prosthesis activates strong braking resistance to knee flexion even when the knee is slightly bent. If the knee is flexed more than 20 degrees, no flexion resistance is provided making stair descent or stumble recovery difficult. Hydraulic non-MPK units provide sufficient resistance to weight bearing knee flexion beyond 20 degrees to allow step-over-step descent of stairs or curbs (Figure 27-2).15 Hydraulic or pneumatic knees also provide variable levels of resistance to knee flexion during swing phase to minimize asymmetry between sound and prosthetic knee flexion at different gait speeds.
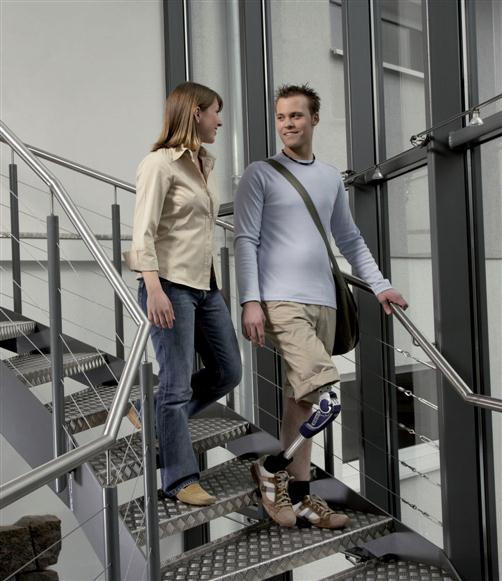
The two different resistance modes in hydraulic knees make these units ideal for those who are able to move at different speeds and traverse a variety of surfaces such as encountered in the community. However, these knees require specific motions during gait to provide the mechanical cue, such as a firm knee hyperextension force of at least 0.1 second in terminal stance phase,11 to switch between the two different levels of resistance required for weight-bearing stance phase and non–weight-bearing swing phase. If a sufficient cue is not achieved at the end of the swing phase, the appropriate resistance to support the weight-bearing limb will not be applied and a fall may occur. Alternatively, if the cue is not achieved at the end of stance phase, the leg may remain stiff in the swing phase, leading to an awkward gait pattern. As a result, the user must be careful to move with adequate hip action to prevent stumbles.
Users of non-MPK prostheses must use compensatory techniques for other activities. For instance, to go from sit-to-stand, the wearer generally places more weight on the sound limb and depends on that leg, and arms as needed, to raise themselves to standing. When sitting, unweighting the prosthetic leg is required in order for the knee to bend easily. Such basic activities place extra stress on the sound limb, which can contribute to the frequent reporting of low back and sound limb pain among prosthesis users.16 Another example is descending slopes, a notoriously difficult activity for users of transfemoral prostheses. A step length matching that of the sound limb often results in a prosthetic knee angle that exceeds the approximately 20-degree safety range of a hydraulic stance phase control or a weight-activated knee unit. Thus, most prosthesis users learn to take very short steps. Finally, ascending stairs step-over-step is very difficult for any transfemoral prosthesis user, generally requiring use of a banister if the step is of standard height.
Introduction to microprocessor knee prostheses
Unlike non-MPK prostheses that use alignment, locked knees, or weight-activated friction brakes, and hydraulic or pneumatic mechanisms, MPK prostheses incorporate an onboard microprocessor to compute data from various electronic sensors and provide real-time adjustments during the user’s activities. The computer’s processor enables rapid adjustments in knee resistance during both swing and stance phases control, usually with pneumatic or hydraulic components. The speed of current microprocessors allows data sampling from sensors in the MPKs at speeds of up to 50 times per second17 to provide more responsiveness to individual movements than can be offered by non-MPK pneumatic and hydraulic knee prostheses. Based on input from various combinations of joint position and motion sensors, pressure sensors, and gyroscopes, proprietary software algorithms determine the phase of gait or function of the leg to provide real-time adjustment of resistance within the MPK unit to facilitate the optimal walking pattern.
The prosthetist performs the initial MPK calibration for the wearer’s typical use patterns with software specific to the MPK manufacturer. Calibration requires that the wearer walk at slow, normal, and fast speeds for approximately 12 m (40 ft). Then the wearer negotiates stairs and ramps so that the appropriate knee resistance levels can be set. Additional adjustments may be necessary as the user bears more weight on the prosthesis and participates in more activities.
MPKs offer a variety of swing and stance phase control functions, including resisted swing-phase knee extension and knee flexion, resisted stance-phase knee flexion, powered stance-phase knee extension, locked or unlocked (free) knee motions, and various combinations for specific functional applications. MPKs offer stance-phase knee resistance within a 0- to 35-degree range (Table 27-1).6
Table 27-1
Microprocessor Knee Prostheses Offer a Variety of Knee Control Functions
Gait-Phase Controlled | Manufacturer | ||||
Endolite | Freedom Innovations | Nabtesco | Otto Bock | Össur | |
Swing only | Smart IP | Intelligent Hybrid | |||
Stance only | Compact | ||||
Swing and stance | Smart Adapt, Orion | Plie 2.0 | C-Leg, Genium | Rheo Knee, Power Knee | |
Stair ascent (powered assist) | Power Knee |
As with the non-MPK units that have both swing- and stance-phase control functions, the MPK must switch between different functions. MPK units receive data from various sensors, such as plantar pressure on different foot regions, that indicate the portion of stance phase, especially initial loading. Some MPKs, like the C-Leg and the Rheo Knee, allow controlled knee flexion upon initial loading to reduce vertical shock impact. Angle and velocity of the knee indicate the oncoming of terminal swing. A MPK like the Genium has a gyroscope, which senses the direction of movement and determines when the user lifts the leg to ascend stairs or to step over an obstacle (Figure 27-3).
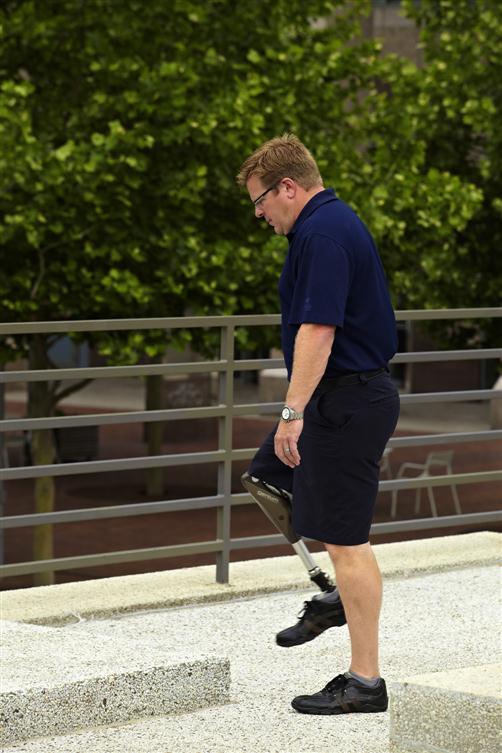
In general, manufacturers suggest that MPKs with stance-phase control be prescribed for Medicare K2- and K3-level users (Table 27-2) whereas MPKs with both stance- and swing phase-control be prescribed for K3- and K4-level users who will utilize different walking speeds.11,17 However, all currently available MPKs are designed for low to moderate impact activities (Table 27-3).11,17 Processor and actuator speeds are insufficient for high-speed activities and, as with all electronic devices, MPKs are vulnerable to overheating. Prosthesis users at the K4 level who engage in high impact activities, such as running or jumping, are more suited to hydraulic non-MPK designs like the Mauch Knee.
Table 27-2
Medicare Functional Levels for People with Unilateral Transtibial and Transfemoral Amputation
Level | Typical User Profile | Functional Abilities with Prosthesis |
K1 | Household ambulator | Has ability or potential to transfer and ambulate on level surfaces at slow speeds with fixed cadence. Time and distance severely limited. |
K2 | Limited community ambulator | Has ability or potential to ambulate and traverse common environmental barriers such as curbs, stairs, or uneven surfaces. Time and distance often limited. |
K3 | Community ambulator | Has ability or potential to ambulate at faster speeds with variable cadence and traverse most environmental barriers. Can undertake vocational, therapeutic, or exercise activity that demands use beyond ambulation. Time and distance still somewhat limited. |
K4 | Active user (child, active adult, athlete) | Has the ability or potential for prosthetic use that exceeds ambulation, including high impact, torsion, or energy levels common to sport. Time and distance essentially unlimited. |
Table 27-3
Levels | Target Activity | Typical Use |
Low | Walking with small cadence variations | Daily walking with low foot forces. Examples: household tasks, gardening, shopping, and occasional nonimpact sports such as golf and leisure walking. |
Moderate | Walking with variable cadence | Daily walking of long durations with moderate forces. Examples: aerobics, jogging, sports like tennis, and vocational activities like lifting/carrying. |
High | High cadence walking | Daily activities involving vigorous and repetitive actions with fast speeds and high loading forces. Examples: distance jogging, running, jumping, sports like basketball, and vocational activities like construction work. |
Sport-Extreme | High impact sports and activities | Daily activities with high or extreme forces common in repetitive, fast, and/or sustained activities. Examples: sprinting, long-distance running, active military service. |
In addition to different combinations of swing- and stance-phase control, the commercially available MPKs have other options. For instance, the Plie 2.0 knee utilizes a pneumatic mechanism that the user pumps regularly to adjust resistance levels (Figure 27-4).12 The pneumatic hybrid is available with both single and multiaxis knee joints that allow up to 160 degrees of flexion.13 MPKs, however, generally provide a knee flexion range from 120 to 140 degrees, which exceeds that of most non-MPKs like the Mauch Knee. MPKs generally dampen knee extension to minimize terminal knee extension impact as well as to adjust the arc of shank swing to the speed of walking, but not early swing phase knee flexion. The C-Leg and Genium have dampened swing phase knee flexion to approximate the 60 degrees normal in level walking.17,18 The Power Knee offers powered robotic assistance in sit-to-stand and stair ascent functions (Figure 27-5).11
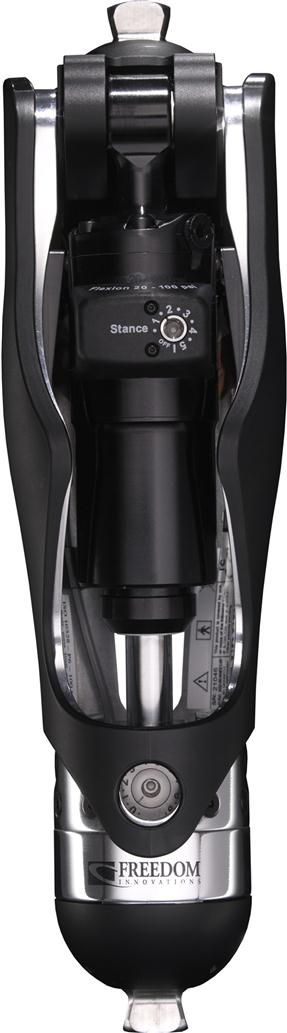
All MPKs have some common characteristics. Although technical specifications of individual MPKs vary, all are powered by batteries that must be charged 4 to 14 hours for use limited in general to 1 to 5 days. The Power Knee, the only MPK to provide robotic assistance to movement, maintains its charge for only 12 hours.11 Depending on use intensity, the Smart Adaptive MPK can maintain charge for up to 14 days.6 The battery and hydraulic mechanisms do not function in all environments and are limited to operating temperatures ranging from − 10 ° C (14 ° F) to 60 ° C (140 ° F) for the C-Leg,17 sufficient for most people’s requirements. As with other electronic devices, such as laptop computers, MPKs are also vulnerable to sand, debris, and water—especially salt water. The most water-resistant MPK, the Plie knee, is purported to be able to withstand occasional submersion in water up to 0.5 m (1.64 ft) in depth (see Figure 27-4).
Electronic signals, such as repeated beeps or vibrations, warn the user of impending shutdown because of computer or hydraulic overload or other malfunction, as well as changes in mode of function. The wearer must learn the meaning of the different signals to assure proper use. Upon shutdown, MPK will default to various states. Most default to swing phase control, which allows knee bending in swing phase but can also permit collapse in stance phase. The C-Leg and Power Knee default to stance-phase resistance, which causes the knee to lock and protects against falls if the microprocessor receives abnormal input such as can occur during a stumble or step onto an obstacle or uneven surface. A stance-phase resistance default setting, however, requires circumduction, hip hiking, or vaulting in swing phase until normal MPK function is restored.
The battery and other electronic components add weight, causing MPKs to be heavier than hydraulic non-MPK units. Weights for MPK units range from 1145 g (2.5 lb) for the C-Leg to 3200 g (7.1 lb) for the more complicated Power Knee; compared to the hydraulic non-MPK units such as the SR9517 at 360 g (12.6 oz) or the Mauch knee which weighs 1140 g (2.5 lb).11 While the Mauch Knee Plus can accommodate high impact use by users weighing to 166 kg (366 lb),11 MPKs are generally designed for low to moderate impact use by individuals who weigh less than 125 kg (275.6 lb). The newly available Genium can support people up to 150 kg (330.7 lb).17 Typical MPK units cost $16,000 to $18,000, with total cost of the prosthesis as much as $50,000 in 2004,19 with 3-year warranties standard; Otto Bock offers an extended 6-year warranty for the Genium.17 Cost can be two to three times the cost of a non-MPK unit. However, in a cost analysis of Dutch transfemoral prosthetic users, total intervention costs were only 23% more because associated rehabilitation expense was less. When patient and family expenses, such as housekeeping and decreased work productivity, were included, the cost to MPK users was roughly half of non-MPK users although the two groups both averaged 47 years of age and had similar work profiles and amputation etiologies.20
Any MPK can be integrated with many other prosthetic components. Endoskeletal construction is typically employed to save weight and provide space for componentry. Each company recommends integrating its MPK with an energy-storing foot selected from its catalog. The difference between feet may not make a substantial difference21 and can be individually determined based on the judgment of the prosthetist, patient, physician, and therapist.
When integrating a MPK into a hip disarticulation prosthesis, some shank, feet, or hip joint units may provide functional benefits. Particularly useful are shank devices that provide transverse plane rotation, such as the Delta Twist, which can dampen over 20 degrees of rotation and can be combined with the C-Leg. The Ceterus foot,11 for instance, also may help provide transverse plane rotation accentuated by the longer step lengths that sometimes result when using a MPK.22 The Helix3D hip joint provides transverse plane rotation unlike other prosthetic hip joints.
Microprocessor knee prostheses control mechanisms
The MPK works by sensors transmitting input to the microprocessor, which converts the data so that the appropriate output can be provided. In some cases, artificial intelligence allows the MPK to adapt to the user’s movements in different activities. Two types of mechanisms provide input to the MPK namely, computational and interactive.23
Computational control mechanisms use sensors to detect movement and forces and send this information to a computer that processes the information and adjusts the resistance provided by the knee mechanism to accommodate for variations determined by the data. For instance, 70% body weight borne through the weight-bearing foot will be interpreted as occurring during stance phase leading to full resistance to knee flexion. This intrinsic mechanism is so-called because the sensory information and decision-making process is intrinsic to the knee unit sensors and microprocessor, which prompts an automatic reaction. It is the most common form of input mechanism.
Interactive control mechanisms, more common to upper-limb myoelectric prostheses, integrate the user’s conscious initiation. Pattern recognition or electromyographic signal sensors detect the movement initiation. Upper-limb prosthetic function is distinctly different from lower-limb function. Arm movement is modulated primarily by the cognitively variable central nervous system to perform complex acts like grasping a variety of foods. In the lower-limb, most everyday function involves walking, which is modulated by the spinal cord and central pattern generators without many fine motor variations.
While the prosthesis user would not want to think about each of the average 6000 steps taken each day,24 the future may bring interactive control of the prosthesis through myoelectric input. Preliminary experiments with people with lower-limb amputations using myoelectric technology in a virtual environment demonstrate that electrodes imbedded in muscles of the residual lower limb can be used to facilitate specific movements, as is typically done in myoelectric upper-limb prostheses. However, the time to complete simple tasks in the experiments, like extending and relaxing the knee in sitting, exceeded 1.5 seconds.25 Perhaps myoelectrically driven intrinsic control mechanisms may eventually assist slow and deliberate non–weight-bearing tasks for people with leg amputation.
Currently, the Proprio foot, outfitted with an accelerometer, joint sensor, and motorized actuator, can plantar and dorsiflex the foot in non–weight-bearing positions upon receiving the correct cues (by heel tap or wireless remote control), enabling the user to sit or don trousers more easily (Figure 27-6). Other functions for the transfemoral prosthesis user, such as rotating the leg to place it on the knee to don shoes and socks, would be the kind of action such an interactive control system may perform in the future.11
Once the sensor data has been input and the microprocessor has determined what function is occurring, the MPK can provide two types of knee movement output: resistance or powered assistance. Most commonly, MPKs resist movement, which can be thought of as an eccentric force. Most knee function in gait is eccentric, whether resisting knee flexion in early stance or resisting knee extension in terminal swing phase. By providing the appropriate amount of resistance through the required range of motion, the MPK can assist the wearer to walk at varied speeds and descend stairs and ramps with less difficulty.
Powered MPKs can also assist movement, comparable to a concentric force. Such a force can be helpful in ascending stairs and rising from a chair, especially for those with bilateral limb loss or a weak intact limb. Although powered assistance provides the potential for the most complete replication of normal leg function, this potential is limited by actuator technology and electromechanical speed. For instance, the human knee moves more than 300 degrees per second in walking26 and can increase to over 600 degrees per second in running.27 It would be difficult for actuators that have activation times only as fast as 10 milliseconds12 to create such high velocities, without overheating when maximum speeds are maintained.
Artificial intelligence is used in MPKs to varying degrees. Standard setup includes initial programmed learning while the wearer walks with the MPK at various speeds and negotiates ramps and stairs. Setup programming prepares the prosthesis for normal function but may not provide sufficient information for the knee to respond appropriately during unexpected events, such as stepping into a divot. Although technically possible, most MPKs do not use real-time accommodation, as it is unnecessary for ordinary use. For instance, even unexpected situations such as stumbles cause predictable inputs that are anticipated by default settings.
Common mobility problems and potential solutions
Despite sophisticated technology, prosthesis users face a variety of problems in moving around the community. Some problems can be significantly improved by MPK use, although users who are transitioning from non-MPK prostheses may have developed habits that must be unlearned. To illustrate how a MPK unit can benefit the user, common problems in gait, stair and ramp negotiation, transfers, and stumbling are presented.
Despite rigorous training and dedicated practice, some gait deviations persist.15,28 The most common deviations that MPKs can significantly benefit are discussed here with prosthetic and training solutions.
Stance Phase
Loading Response
A common stance-phase deviation is decreased prosthetic knee flexion during loading response. Decreased knee flexion develops because amputation of the knee robs the lower limb of the eccentric function of the quadriceps, which typically absorbs impact shock as the knee flexes approximately 15 degrees during initial loading.29 Knee buckling in loading response is a primary concern in early prosthetic training. The experienced non-MPK user prevents collapse and potential falls by keeping the knee extended in loading response. Decreased prosthetic knee flexion, however, diminishes shock attenuation and transmits stress up the kinetic chain to the hip, pelvis, and spine.30 Weight-activated friction brakes stabilize the knee when in the safe 0 to 20-degree knee flexion range. Hydraulic units provide graded resistance to knee flexion within the 0 to 20-degree range for descent of stairs, but will buckle readily beyond this range. Although this range of support is usually adequate for level walking, more range is required when descending a steep ramp, stepping on uneven surfaces, or when missteps occur. Lacking graded eccentric knee flexion control upon initial loading, the user learns to walk with a habitually extended knee.
Prosthetic Solutions
Some MPKs, like the C-Leg and Rheo Knee, allow knee flexion upon loading to provide the normal shock-absorbing function of the anatomic knee upon heel strike. For experienced prosthesis users who have learned to walk with the prosthetic knee extended upon initial contact, this function may seem strange. Indeed, the transfemoral amputation limb generates substantial hip extension power in the initial loading phase of gait particularly on the amputated side to push the thigh posterior and maintain the knee extended as well as to power the body forward over the stance limb.31 The new MPK user transitioning from a non-MPK unit must unlearn old habits and let the knee bend upon initial contact to benefit from the MPK’s capacity for greater shock absorption. The prosthetist can adjust the level of resistance as the user adapts.
Training Solutions
Whether learning to walk with a prosthesis for the first time or transitioning to an MPK that allows dampened knee flexion upon initial contact, prosthetic training should develop both movement ability and trust in the leg. Although strengthening gluteus maximus is always beneficial to increase eccentric motor control that can support knee flexion control, the major factor is developing the trust in the MPK to allow knee flexion. Initially standing in parallel bars to provide security, the MPK user can step forward onto the prosthesis, perceiving the resistance to knee flexion as weight progresses from the heel to the toe. Repeatedly leaning on the prosthetic foot to rock from heel to toe as the knee bends gives the MPK user awareness of the strength of knee resistance and helps foster trust in the leg (Figure 27-7). Training can progress to practice stepping performed with knee flexion upon heel contact as the body advances over the prosthetic foot causing knee extension, similar to able-bodied gait. This can be practiced in the parallel bars and later advanced to walking with initial knee flexion upon heel contact, guarded by the physical therapist who can insure that the knee unit will progress into extension as in normal gait. Training proceeds to ramp descent—best begun using a railing with therapist assistance. Developing the confidence to descend ramps while the MPK flexes through initial loading can seem like a leap of faith at first. Making the transition from walking with a hyperextended prosthetic knee to allowing the knee unit to flex during loading response can be difficult. Nevertheless, as few as 10 weeks has been needed to acclimate to the MPK.32
Asymmetric Step Length
Various physical impairments make asymmetric step length a common deviation for prosthesis users. The sound limb step is typically shorter than that of the prosthesis. Amputated side hip extensor weakness, uncertain balance, and limited hip extension range of motion, further restricted by the 10-degree hip flexion built into the transfemoral socket bench alignment, all cause a briefer sound limb swing time and shorter step length. Decreased hip rotation and concomitant lessened contralateral pelvic rotation also contribute to shorter prosthetic steps. Increasing hip extension range of motion and hip strength improves balance and facilitates longer prosthesis stance time. Practice walking with shorter sound limb step lengths can also reduce asymmetry.22
Gluteus maximus strength is critical during initial loading to generate the hip extension force in early stance that lifts the center of gravity from lowest to highest point and converts stance limb torque from internal to external rotation. Extensor strength is the strongest predictor of prosthetic walking speed.33 In the absence of quadriceps and with the inevitable atrophy of the hamstrings,34 hip extension and abduction display the greatest strength loss after amputation.35 Atrophy of gluteal fast twitch fibers explains the slower gluteal contraction latency periods observed in amputated limbs.36 Greater demand and slower contractions on the weakened amputated side hip extensors decrease the user’s ability to quickly raise the center of gravity from the lowest point in dual limb stance to the highest point by midstance,33 particularly if long prosthetic steps are emphasized early in the rehabilitation process when gluteal strength is weakest. As a result, prosthetic stance time is significantly briefer than on the sound side, leading to shorter sound limb swing phase duration and step lengths.37,38
Hip abductor weakness reduces the ability to maintain the body in prosthetic limb stance. This leads to a similar scenario in the frontal plane that also contributes to shorter sound limb steps.39 Insufficient gluteus medius strength also diminishes the confidence to maintain single limb stance long enough to complete the normal lateral weight shift. The prosthesis user compensates by placing the sound foot farther from the midline, widening the base of support. The wide base shortens the gluteus medius length–tension relationship, further impairing hip abduction strength while simultaneously requiring a larger lateral weight shift. Hip abductor weakness also plays a role in step-length asymmetry, with weakness correlating with slower gait, shorter steps on both sides, and decreased weight bearing on the prosthesis.39 Those with shorter amputation limbs have more abductor weakness, demonstrated in midstance by faster and/or greater pelvic drop.40,41 In both situations, longer and/or wider steps disadvantage the gluteal muscles and can undermine balance confidence.
In terminal stance, decreased prosthetic side hip extension restricts the body’s advance over the prosthetic foot causing the sound limb to take a shorter step forward. Lack of sufficient hip extension because of hip flexor contracture occurs in able-bodied people but is more prevalent among people with lower-limb amputation. Prolonged sitting during the rehabilitation process that can continue at home because of decreased activity is common after amputation.24 The standard flexed bench alignment of the transfemoral socket can accommodate mild hip flexion contractures but reduces hip extension excursion.38
In the presence of limited hip extension range of motion, users attempt to advance the body over the prosthesis by exaggerating anterior pelvic tilt40,41 with accentuated lumbar paraspinal muscle use, leading to greater lumbar extension compared to able-bodied people.42 Such compensation may lead to lower back strains; people with both amputation and low back pain had weaker back extensors.43 Increased demand for hip and lumbar extension strength and range of motion might be met with extra training to guard against low back pain. Abdominal and hip strength is also critical to protect end-range lumbar extension as well as to assist atrophied hip flexors.44
In normal gait, stance phase hip extension occurs with rotation around the stance hip.29 Although often observed as contralateral forward pelvic rotation, the rotation occurs primarily at the hip. After amputation, hip extension and contralateral forward rotation around the prosthesis are greatly reduced compared to the sound limb,40 because transection through the femur minimizes transverse plane bony leverage. As a result, translation of rotary forces from the limb to the socket is greatly reduced because the femur rotates within the soft tissues of the thigh. Any looseness in socket fit reduces the translated forces even more. In fact, unlike sound side or able-bodied individuals, prosthetic stance phase is marked by internal, rather than external, torque,41 which decreases trunk counterrotation and arm swing (Figure 27-8). Less trunk rotation is needed to counterbalance pelvic rotation when the individual wears a prosthesis. Nevertheless, when pelvic rotation is decreased, trunk rotation for prosthesis users is also diminished by limited joint mobility, weaker abdominal strength, incoordination between pelvis and trunk, and habit.
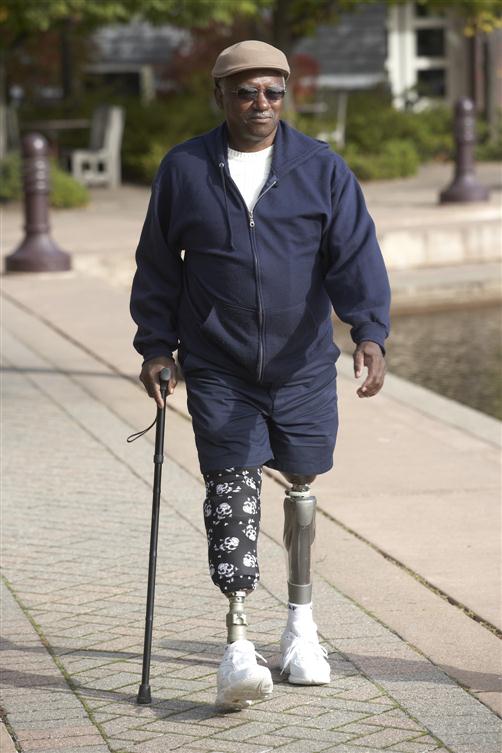
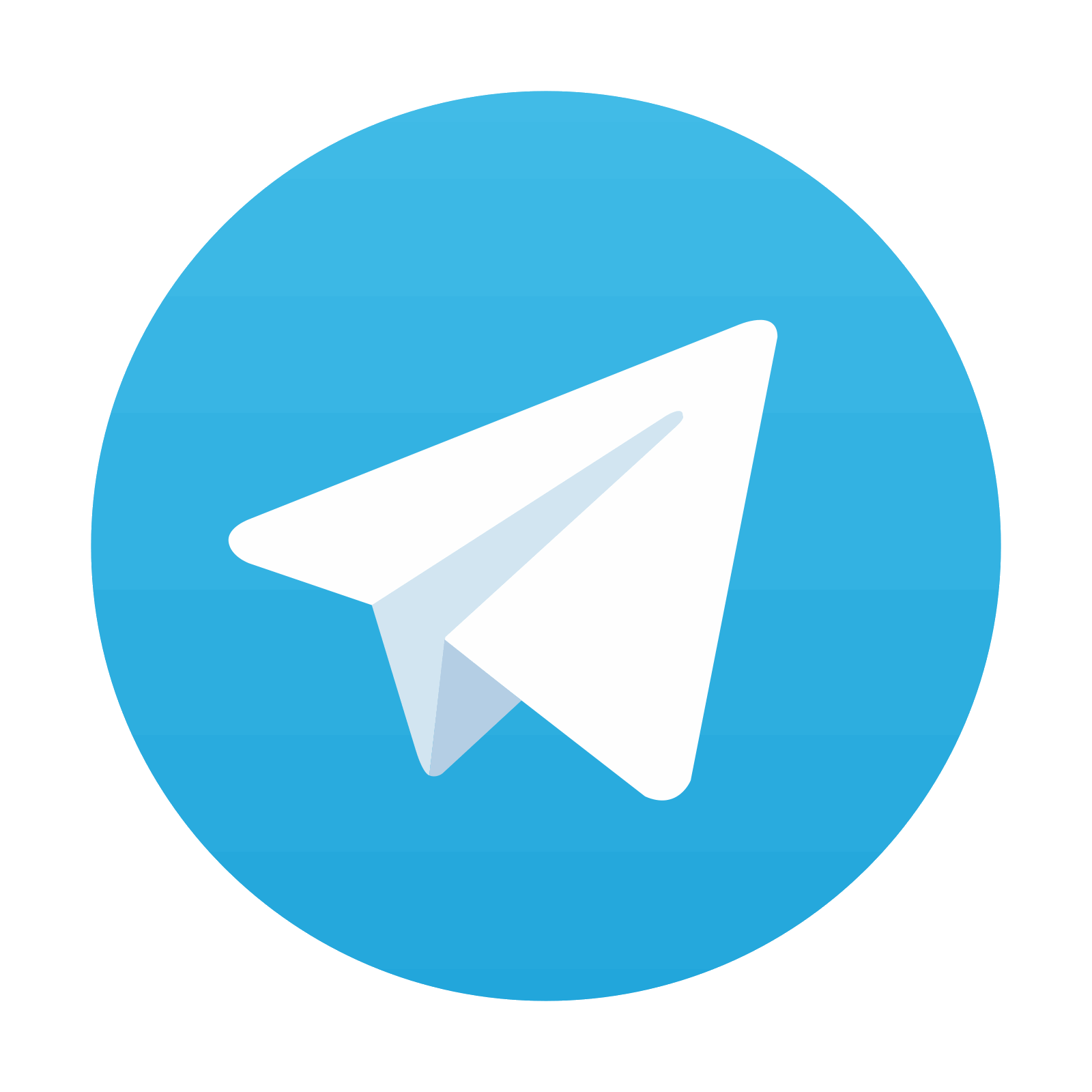
Stay updated, free articles. Join our Telegram channel
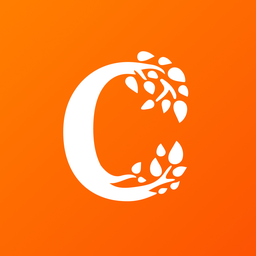
Full access? Get Clinical Tree
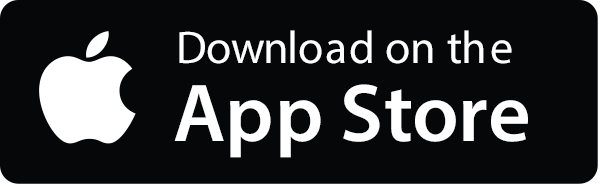
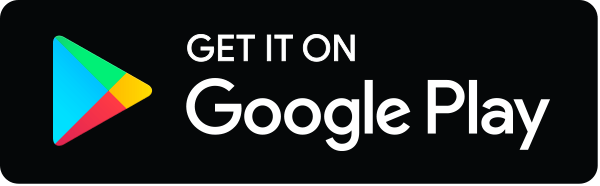