Toward Engineering a Biological Joint Replacement
Over 20% of the adults in the United States (25 years and above) have osteoarthritis (OA) of the hip or knee (>46 million Americans).1 OA is ranked as one of the top three causes for disability and contributes more than $185 billion dollars a year in related medical costs.2–4 More than 580,000 arthroplasty procedures are performed each year in the U.S.5 While joint replacement generally succeeds in decreasing or eliminating pain and restoring joint function, the lifespan of prostheses is limited due to wear, loosening, infection, and fracture of the implant or surrounding bone.6–9 Alternative treatments have not yet been successful in providing a viable long-term option for cartilage repair. For example, allografts are limited by donor tissue availability and graft viability,10,11 while autografts are limited by the availability of healthy tissue and donor site morbidity. Bioengineered repair strategies that circumvent these limitations, while preserving the natural function of the joint and using a procedure less invasive than total joint arthroplasty, may be optimal for treating younger OA patients.
Articular cartilage serves as the load- bearing material of joints and possesses excellent friction, lubrication, and wear characteristics.12 Successful replacement of damaged or injured articular cartilage will hinge on the ability to recapitulate the mechanical and structural properties of the healthy native tissue before implantation. Over the past two decades, there has been a wide interest in developing functional engineered cartilage. To grow cartilage tissue, cells are cultured within a three- dimensional (3D) scaffold that provides an initial structure for the de novo tissue13–15; alternatively, cells may be cultured using scaffold-less techniques.16,17 For example, autologous chondrocyte implantation (ACI) is a cell-based strategy in which cells are injected directly into focal lesions and covered with a periosteal flap,18,19 whereas CARTIPATCH18 (TBF Tissue Bioengineering, Bron, France) uses a 3D agarose hydrogel scaffold to prevent leakage of cells, stabilize the chondrocyte phenotype, and promote a homogeneous distribution of cells.18 Although these techniques are designed for repair and regeneration of cartilage focal lesions, they can be scaled up to replace an entire articulating layer ( Fig. 15.1 ). However, nutrient diffusion through the depth of these large scaffolds represents a major challenge facing the field.
There are two prevailing points of view regarding implantation of engineered cartilage constructs; one approach places the cell–scaffold construct immediately into the defect site and relies on the in situ biological and loading environment to foster construct development (e.g.,20–27). Using this approach, poly -caprolactone (PCL) scaffolds have been designed to provide sufficient mechanical support upon implantation, while being porous enough to permit de novo tissue development.26,27 Lee et al demonstrated tissue growth in a full surface repair of a rabbit proximal humeral head following implantation of an acellular PCL scaffold infused with transforming growth factors.27 Scaffolds that provide sufficient mechanical support at implantation reduce the need for extended culture periods before the repair surgery. The regenerated tissue develops under physiological loading conditions, which may ideally provide better functional tissue.

Another approach is to first precondition the cell–scaffold construct in vitro before implantation into the defect (e.g.,28–32). In vitro cultivation provides a controlled nutrient supply and loading environment that may be optimized for matrix synthesis to produce stiff cartilage-like constructs that may ideally sustain physiological loading following implantation. The required mechanical properties of the engineered cartilage will be dictated by the extent of the damaged region and its mechanical demands. In this type of approach, studies have demonstrated that the most robust tissue properties may be achieved by optimizing the media formulation as well as the transport of solutes in the developing tissue. Applied dynamic deformational loading to cell-seeded hydro-gel constructs provides physical cues to cells and enhanced solute transport, leading to improved mechanical properties compared with free-swelling (unloaded) control.14,24,33–39
Articular cartilage is a highly hydrated soft tissue whose solid organic matrix is composed mostly of collagen fibrils (10 to 20% mass by wet weight) and proteoglycans (5 to 10% mass by wet weight).40–44 Chondrocytes compose less than 10% of the tissue volume45 and maintain the tissue by synthesizing and secreting extracellular matrix. Chondrocyte morphology and biochemical and mechanical properties vary through the depth of the tissue. Near the articular surface cells are more elliptical, and the tissue is softer than in the middle and deep zones.46–49 There have been many improvements in biological replacement strategies for cartilage; however, there are still some limitations and challenges that remain to be addressed for successful repair and regeneration.50 The purpose of this review is to summarize our advances in engineering cartilage and to identify approaches for scaling up these strategies to engineer large constructs suitable for replacing entire articular surfaces in cases of traumatic injury and advanced joint degeneration.
Cell Sources for Cartilage Tissue Engineering
Previous studies have been successful in cultivating functional engineered cartilage using cells from juvenile bovine and adult canine cartilage.24,51,52 These studies have reported equilibrium compressive mechanical properties and glycosaminoglycan (GAG) content similar to native values.25,35,36,53 Cultivating functional tissue with adult chondrocytes shows promise for using these techniques as a clinical repair strategy, since OA is commonly observed in older patients. Autologous chondrocytes from a nearby healthy region of the joint are the ideal cell source for clinical applications of engineered cartilage. However, acquiring enough cells while minimizing donor site morbidity remains a major challenge. Donor cells can be expanded in culture before implantation, but the proliferation rate of human adult cells is generally low and would lengthen the time between surgeries.
Alternatively, stem cells have been investigated as a possible cell source for cartilage regeneration.26,30,51,54–58 Mesenchymal stem cells (MSCs) from adipose tissue, bone, and synovium express surface markers also expressed by chondrocytes, suggesting that MSCs can potentially differentiate toward a chondrogenic lineage.55,59–61 Cells can be encouraged toward chondrogenesis by using a cocktail of growth factors to expand the cells in culture.54,55,57,62 Including growth factors, such as basic fibroblast growth factor (FGF), platelet-derived growth factor (PDGF), and transforming growth factor (TGF) in the expansion culture medium improves the mechanical and biochemical properties of engineered cartilage in 3D culture.55 Expansion of MSCs in vitro reduces the amount of tissue required to obtain a sufficient number of cells, mitigating damage to healthy tissue. Furthermore, encapsulation of MSCs in hydrogels and woven scaffolds demonstrates that these cells are capable of producing cartilage-like tissue with mechanical and biochemical properties tending toward native cartilage values ( Fig. 15.2 ).26,30,55

Previous studies that have used bone- or synovium-derived MSCs have demonstrated that these cells can be passaged and expanded in monolayer culture with a medium defined to promote chondrogenesis.51,55,62,63 In contrast, culturing chondrocytes on a hard surface, such as a glass plate or tissue culture flask, causes the cell to dedifferentiate and become more fibroblast-like. The change in cell behavior may be beneficial for increasing collagen production in vitro. A recent study by Anderson and Athenasiou demonstrated that passaged chondrocytes can self-assemble into engineered cartilage plugs and that passaged cells produce two times more GAG and five times more collagen than primary chondrocytes.64 However, most of the collagen produced with passaged cells was type I collagen, which is not ideal for recapitulating the native cartilage tissue composition.64 In contrast, culturing differentiated stem cells in a 3D scaffold that promotes chondrogenesis (i.e., hydrogels) improves collagen type II production.63 The loading environment experienced by the cell in two-dimensional (2D) culture significantly affects the matrix produced by the cells in 3D culture.55,64
Strategies for Improving Nutrient Diffusion: Enhanced Transport of Nutrients
Closer examination of engineered cartilage constructs reveals that the mechanical properties and matrix distribution are spatially heterogeneous. These constructs typically exhibit mechanically stiffer regions and greater matrix deposition near their peripheral boundaries, whereas regions deeper inside the constructs are typically softer, showing less matrix deposition.65 Nutrient diffusion deep into the construct is a challenge in the field that will be considerably amplified when thicker and wider constructs are cultured. Human articular cartilage can be up to 7 mm thick66,67; therefore, thicker constructs will be necessary for clinical applications.
Immature articular cartilage is well vascularized with canals that provide nutrients to the developing tissue and remove waste by-products.68–71 Experimental studies have demonstrated that dynamic loading enhances the uptake of nutrients into agarose hydro-gels and immature cartilage.72,73 For smaller macromolecules (i.e., 3 kDa), uptake by cartilage under dynamic loading conditions was threefold higher than uptake by passive diffusion. The effects of dynamic loading were found to be more pronounced with larger macromolecules. A 70 kDa molecule achieved a concentration nine times higher under dynamic loading than under passive diffusion into cartilage. This enhanced uptake of nutrients by the tissue was considerably facilitated by the network of cartilage canals, allowing for nutrients to transport deep into the tissue ( Fig. 15.3 ). The increase in nutrient uptake was attributed solely to dynamic loading by demonstrating a return of the solute concentration to passive diffusion levels after termination of loading. Thus, loading provides an active solute-pumping mechanism because the solid matrix of the immature cartilage can impart momentum to the solute at the tissue–bath interface, pulling it into the tissue.72,73

Similarly, short-term dynamic loading of engineered cartilage constructs (i.e., for less than 3 hours) has demonstrated improved nutrient diffusion.33,34,74 Loading of anatomical-size patellar constructs doubled the concentration of large molecules (70 kDa) in the constructs compared with constructs under free-swelling conditions (control; Fig. 15.4 ).34 Dynamic loading has been shown to significantly improve mechanical properties of engineered cartilage, suggesting that the increased nutrient uptake during the 3 hours of daily loading influences matrix production and deposition.24,34,36,74,75 The loading type, duration, and frequency can greatly impact the mechanotransduction response of chondrocyte-seeded scaffolds.74–76 Long-duration loading protocols (6 hours) result in a decrease in the nutrient diffusion into large constructs during loading, primarily due to decreased surface area available for free diffusion.34 However, these constructs produce stiffer engineered cartilage than constructs cultured under free-swelling conditions.75 In contrast to the beneficial effects of dynamic loading, static loading significantly decreases the nutrient uptake by engineered cartilage ( Fig. 15.4 ). These findings in engineered cartilage are consistent with the observation that dynamic loading produces enhanced uptake of solutes into agarose and cartilage,72,73 considerably greater than under passive diffusion. However, other mechanotransduction pathways may also be at work when constructs are being loaded dynamically. Although the precise nature of these mechanisms is not completely understood, dynamic loading can be used to improve nutrient transport into large constructs designed to replicate entire articular layers such as the human retropatellar surface ( Figs. 15.1 and 15.4 ).
Perfusion, a convective transport method, applies a biomimetic approach to provide nutrients into engineered constructs by mimicking the function of the vascular canals in developing cartilage ( Fig. 15.4b , c ). Currently, there are conflicting findings in the literature for the beneficial effects of perfusion, which may suggest further research is needed to determine the optimal flow rate and duration, and when perfusion should be applied over the tissue maturation period. Raimondi et al demonstrated that perfusion of chondrocyte-seeded constructs can potentially improve cell viability, GAG synthesis, and mechanical properties.77,78 Grayson et al demonstrated that perfusion of nutrients through the bone region of an osteochondral construct improves matrix production and distribution in the engineered cartilage.79 In contrast, studies that have combined perfusion with dynamic loading have not observed additional nutritional benefits from perfusion.34,78,80 The findings of these studies suggest some potential benefits of using perfusion in the absence of mechanical loading stimuli to improve the compositional and mechanical properties of immature osteochondral constructs. This will be especially important for large osteochondral constructs because the bone–substrate interface will make limited nutrient diffusion into constructs an even greater challenge to overcome.

Strategies for Improving Nutrient Diffusion: Designing Multiscale Nutrient Pathways
Inspired by the anatomy and physiology of developing native tissue, microscopic and macroscopic channels have been incorporated in engineered cartilage constructs81–85 to provide pathways for improving nutrient transport. Large vascular-like canals can be incorporated at the macroscopic level by creating one or more channels through the thickness of the scaffold during fabrication. Adding a macroscopic channel (1-mm diameter) in the center of a cylindrical hydrogel construct (4-mm diameter) is a very effective method for decreasing the nutrient path length and improving the depth-dependent mechanical properties over time in culture ( Fig. 15.5 ).81 Over time in culture, chondrocytes located in proximity to the channel deposit extracellular matrix that progressively fills it. Thus, even though channels may improve nutrient supply only initially, they may be most beneficial in the formative stages of large engineered constructs and may not be as critical for maintaining tissue properties following implantation into a joint. This nutrient channel method may be scaled up for larger scaffolds by adding more channels. In a study of 10-mm-diameter constructs, the placement of three channels produced tissue with mechanical properties similar to native cartilage.81 As cartilage tissue engineering moves toward cultivating biological replacements for the entire articular surface, an array of channels may be critical for achieving adequate mechanical and biochemical properties.


Another approach that is being investigated is to incorporate lipid-shelled microbubbles or microtubes as a porogens for hydrogel scaffolds.85–88 Originally designed for drug delivery,89–91 these biocompatible porogens are utilized directly with cells during the hydrogel scaffold cross-linking process. This allows engineers to create microlevel porosity in the superstructure of the hydrogel, while maintaining tight, nano-level porosity in the scaffolding directly around the embedded cells. The porous superstructure creates pockets of fluid–fluid nutrient reservoirs that provide less resistance to solute diffusion ( Fig. 15.6a ).88 Preliminary studies suggest that microporogens improve the homogeneity of mechanical properties through the depth of the construct.81,88 Constructs with a relatively low concentration of microporogens (0.2% wet by volume or 10% of the agarose hydrogel concentration) are more opaque and are two times stiffer than control constructs without microporogens ( Fig. 15.6b ).85,88
The gas-filled lipid microbubbles are incorporated into the scaffold filled with a stable gas. The size of the microbubbles and the gas that is used to create them can be altered to control parameters such as the dispersion rate of the bubbles and the porosity of the hydrogel. Intriguingly, it may be possible to create microbubbles that maintain their gas phase for extended periods of time. Under this scenario, it may be possible to purge the gas (and thereby create a fluid-filled pocket) later in culture. This would allow both spatial and temporal control of hydrogel porosity. It may even allow platen-less dynamic deformational loading as the gas phase of the bubbles is utilized in a hydrostatic pressure chamber.
Alternatively, lipid microtubes provide a hard tubular shell that may act as a nutrient channel on the microscopic scale (diameter 0.5 µm, length = 40 µm).92 Similar to the lipid-microbubbles, preliminary data suggest that these porogens can be incorporated into hydrogels to improve nutrient diffusion into engineered constructs. Moreover, the length of the micro-tubes can be increased to provide larger fluid-filled pockets. This approach combines the decreased nutrient path length provided by channels and the enzyme or nutrient loading capability of microporogens. Since micro-tubes have a lipid wall between the cells and the open channel, they are not expected to fill with extracellular matrix with time in culture, providing long-term enhanced nutrient diffusion. Although these studies have shown promise for using microtubes to increase the scaffold porosity, nutrient diffusion, extra-cellular matrix production, and mechanical properties,85,87,88 future work is needed to confirm that the micropores are maintained with long-term culture and with physiological levels of loading.
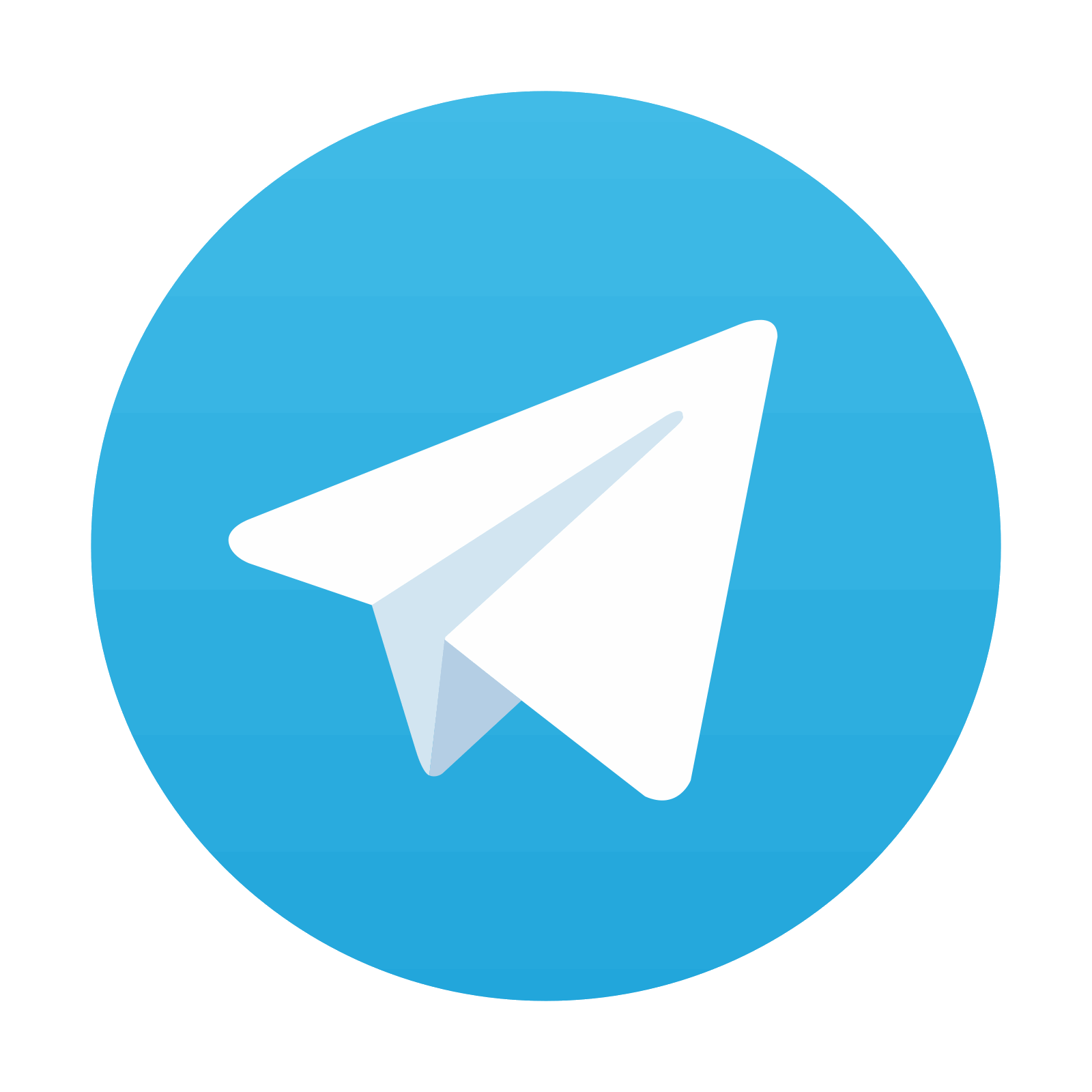
Stay updated, free articles. Join our Telegram channel
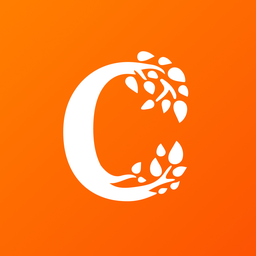
Full access? Get Clinical Tree
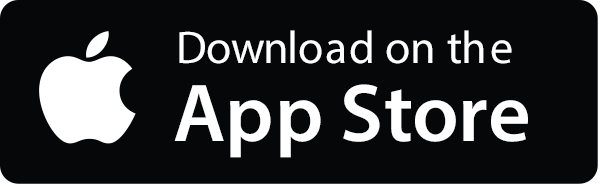
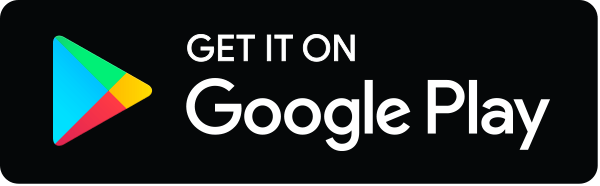