Science and Animal Models of Marrow Stimulation for Cartilage Repair
Microfracture perforation of the subchondral bone for cartilage repair was originally described by Steadman in 1994.1 Microfracture involves penetration of the subchondral bone plate with an arthroscopic awl to allow bone marrow contents to fill the defect and form a “superclot.”2 In humans1,3,4 and nonhuman primates,5 microfracture results in increased tissue volume and improved patient comfort and function for an average of 2 to 3 years. There are other described methods of bone marrow stimulation such as drilling and abrasion, but less research and clinical data are available to critically evaluate the efficacy of these techniques.
The Superclot
In theory, enhanced cartilage repair following microfracture is the result of the superclot thought to be laden with bone marrow-derived mesenchymal stem cells (MSCs) and growth factors.6 Although there have been several in vitro and in vivo animal studies aimed at understanding how micro-fracture repair tissue remodels over time, it has never been well documented that the superclot contains MSCs or growth factors. In a small study of 11 human patients with fem-oral condylar defects, superclot from micro-fracture was compared with bone marrow aspirate from the iliac crest and concentrated by centrifugation.7 The two cellular populations were different with respect to cell surface markers. Neither cell type carried CD34 or CD45 marker expression, suggesting that there were no hematopoietic cells in either bone marrow aspirate concentrate or micro-fracture superclot. This result might suggest that neither cell source is derived from the bone marrow, but it must be interpreted with great caution because both cell sources were cultured for at least two passages and the cells were treated with trypsin before flow cytometry analysis, both of which have been documented to alter cell surface protein expression on stem cells.8,9 In a similar study in which cells were derived from subchondral corticospongious bone and cultured over time, the cells retained their multilineage potential to undergo trilineage differentiation into cartilage, adipose, and bone phenotypes.10 Interestingly, MSC-based cartilage studies continue to focus predominantly on the ability of the cells to differentiate into and form neocartilage despite the growing evidence that MSCs function at least in part to modulate the local environment through a paracrine effect and recruitment of other progenitor cells and immunomodulation.11,12
Understanding the source and type of cells that populate microfracture defects is critically important. There are a number of studies that have evaluated the various effects of drugs, growth factors, devices, scaffolds, gene therapy, and rehabilitation on microfracture. Some of these modifying factors are being promoted and even marketed predicated on the concept that they enhance chemo-taxis, adherence, and/or proliferation of bone marrow-derived MSCs.13–19 These cited studies represent only a few of the many studies investigating the use of scaffolds, devices, and drugs, in vitro and in rabbit, canine, ovine, laprine, or equine animal models for augmentation of microfracture to enhance articular cartilage repair. This intense level of investigation into scaffold/device- augmented microfracture and its potential recruitment of MSCs lies in the thought that these technologies could improve the clinical results of microfracture alone and the relative ease and marketability of such technologies when compared with cultured or manipulated stem cell articular cartilage grafts.
If the cell population of the subchondral bone is truly different from that of bone marrow aspirated from a bone marrow space, then perhaps the results of in vitro studies done on bone marrow aspirate or metaphyseal-derived MSCs are not directly applicable to microfracture, in which the cell is likely derived from the subchondral bone plate in the area 2 to 4 mm underlying the calcified cartilage layer.13,15 During the process of maturation, the cell population in a superclot might be composed of cells derived from the bone marrow, subchondral bone, surrounding host cartilage, synovium, syno-vial fluid, or a combination thereof. Studies are routinely performed in vitro, and using bone marrow-derived MSCs to investigate a method to improve microfracture and the results can change clinical practice. For example, a recent study showed that chondrogenic differentiation of bone marrow-derived MSCs is impaired by rheumatoid arthritis synovial fluid as compared with synovial fluid from patients with osteoarthritis or normal patients.20 Another study suggested that age in males, but not in females, negatively affects their ability to undergo chondrogenic differentiation.21 The potential clinical ramifications of this study, where clinicians might presume failure of microfracture in patients with rheumatoid arthritis or in older males, underscore the need for a more refined understanding of the basic biology of microfracture.
Animal Model Studies
Animal model studies provide insight into temporal changes following microfracture ( Fig. 6.1 ). Early animal model studies on microfracture repair were done in the horse.22,23 The horse model was also used to validate the subjective clinical impression that removal of the calcified cartilage layer was important to optimize volume and attachment of repair tissue.24 Further equine studies indicated that the volume of repair tissue did not change between 4 and 12 months postmicrofracture in direct weight-bearing sites (distal medial femoral condyle and distal radiocarpal bones), which at a minimum suggests that the repair tissue did not deteriorate by 12 months postoperatively.22 Histologic assessment revealed that there was more type II collagen present at 12 months than at 4 months, suggesting continued chondrogenic maturation of repair tissue to 12 months, but the aggrecan content remained far below normal.
To provide information in a physiologic and anatomic environment more closely related to the human, similar studies were performed in cynomolgus macaques.5 In this study, repair tissue was studied at 6 and 12 weeks postmicrofracture and indicated that the repair tissue underwent progressive chondrogenic remodeling during this time period based on postmortem gross and histologic assessments. It is interesting to note that progressive maturation of microfracture repair tissue is not appreciated using arthros-copy with validated categorical scoring systems,25 which makes it difficult for a surgeon to make decisions regarding success based on arthroscopic observation only.26 Noninvasive dGEMRIC and T2 mapping has been used to evaluate repair tissue following microfracture at 24 and 48 weeks postoperatively in a goat model.27 The achieved objective of the study was to validate dGEMRIC and T2 mapping as surrogate markers of biochemical and histologic integrity of repair tissue. In addition, the study was the first to demonstrate increased glycosaminoglycan and total collagen content between 24 and 48 weeks postmicrofracture measured with both ∆R1 (1/s) and high- performance liquid chromatography. Combined, these results suggest that microfracture continues to mature for the first 12 months after surgery, but the lack of normal matrix molecules translates to tissue with inferior biomechanical properties compared with normal cartilage, which renders the repair tissue prone to injury and deterioration. Based on animal model studies, it is unclear what biochemical or mechanical changes happen beyond 12 months and when, why, or how microfracture repair tissue fails or not. In unpublished data by L.A.F, 2-year data are being analyzed in the horse. Clinically, it may have less to do with the breakdown of microfracture repair tissue than with ability of the repair tissue to “shield” the subchondral bone from load that is theoretically associated with the manifestation of symptoms. If this theory is correct, then methods to enhance or retain proteoglycan content in the repair tissue would increase the compressive stiffness of the repair tissue and should improve long-term results.

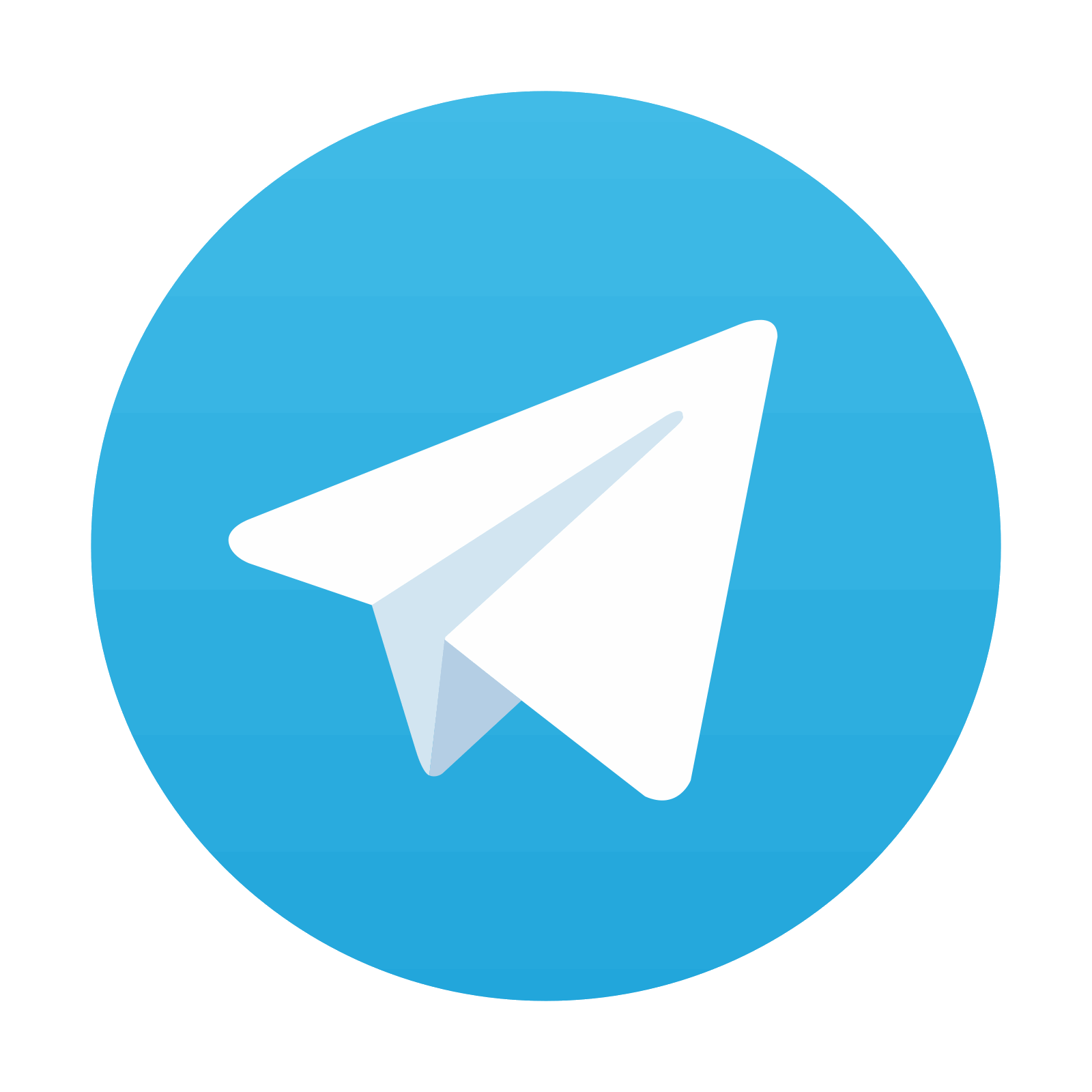
Stay updated, free articles. Join our Telegram channel
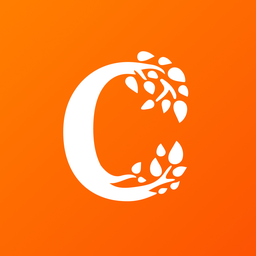
Full access? Get Clinical Tree
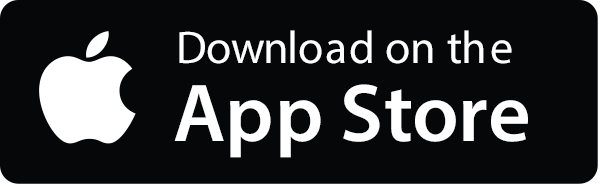
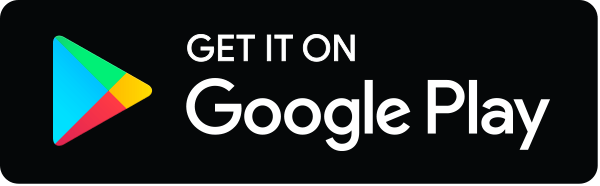