© Springer Japan 2016
Shin’ichi Takeda, Yuko Miyagoe-Suzuki and Madoka Mori-Yoshimura (eds.)Translational Research in Muscular Dystrophy10.1007/978-4-431-55678-7_99. Therapeutic Approach of iPS Cell Technology for Treating Muscular Dystrophy
(1)
Department of Clinical Application, Center for iPS Cell Research and Application (CiRA), Kyoto University, 53 Shogoin-Kawahara-cho, Sakyo-ku, Kyoto 606-8507, Japan
Abstract
Most genetic disorders of skeletal muscle do not have curative treatment and are limited to palliative care that fails to curb progression. Additionally, for many myopathies, the pathology remains unclear, although the causative genes have been identified. Thus, research attempts are underway to develop new treatments for these intractable myopathies, including those based on induced pluripotent stem (iPS) cells. In general, iPS cell strategies can be divided into two groups: those focused on cell transplant therapies in the hope of finding a curative treatment and those seeking to elucidate the underlying pathology to develop effective drugs.
The transplantation of muscle progenitor cells derived from mouse iPS cells promotes muscle regeneration in a muscular dystrophy mouse model and restores muscle strength. Similarly, research using human iPS cells has led to several methods for inducing muscle engraftable progenitors. Though reports on pathology studies using patient-derived iPS cells are few, they have shown successful recapitulation of the disease. Here I explain how the induction of differentiation of human iPS cells into muscle cells with exceptional efficiency and high reproducibility will boost drug development and therapeutic efforts along with providing detailed understanding of myopathies.
Keywords
iPS cellCell therapyDisease modelingDrug screening9.1 Introduction
Pluripotent stem cells are capable of considerable proliferation and are theoretically able to differentiate into any kind of somatic cell, which gives them promise as a source of cells for various transplant therapies. Induced pluripotent stem cells (iPS cells) are pluripotent stem cells that were derived by introducing and expressing specific genes into somatic cells [1, 2]. They share many properties with another pluripotent stem cell, embryonic stem cells (ES cells), but have two significant advantages: (1) in transplantation therapy, the rejection reactions can be much lower since iPS cells are derived from patient’s cells whereas ES cells come from another source, and (2) iPS cells have fewer ethical concerns since fertilized eggs are not destroyed in the process. Already, research on iPS cells in cell transplant therapies is being carried out for many intractable diseases such as age-related macular degeneration [3] and spinal cord injuries [4].
There is now a concerted effort to expand the use of iPS cells in cell transplant therapies to skeletal muscle diseases, including intractable myopathies such as muscular dystrophy. Currently, satellite cells, which are in vivo skeletal muscle stem cells, are considered the best source of cells for transplant therapy [5, 6]; however, current culturing methods cause satellite cells to differentiate into myoblasts, which have limited regenerative ability and therefore compromises their use for cell transplant therapy [5]. Alternatively, there has been an effort to transplant the embryonic origin of satellite cells, namely, muscle progenitor cells, into host skeletal muscle and then induce their differentiation into normal satellite cells in vivo. ES [7] and iPS cells are recognized as potential sources for generating muscle progenitor cells. If ES/iPS cell-derived muscle progenitor cells could form satellite cells and repeatedly regenerate and proliferate when transplanted into the skeletal muscle, effective muscle regeneration would be possible even with only few transplants.
Another therapeutic approach using iPS cells is drug screening and drug development. Because iPS cells are derived from patients with intractable diseases, inducing them to differentiate into a specific cell type, i.e., the diseased cell type, offers a new human model that reproduces the pathology in vitro for drug testing [8]. To date, knockout mice have been used as primary disease models; however, knocking out human orthologous genes in these models often fails to reproduce the disease pathology. Further, drugs developed using mouse models are too frequently ineffective in humans. Consequently, pathology models constructed from human cells are quintessential for future drug development. Presently, myoblasts from Duchenne muscular dystrophy (DMD) patients are the most common cell source for modeling the pathological condition [9]. Yet DMD patient myoblasts show certain phenotypes that make them unreliable for disease modeling. For example, patient skeletal muscles vary in nature from individual to individual depending on various factors such as genetic background, age, and medical history. Furthermore, muscle cell differentiation from DMD patient myoblasts is delayed and has poor proliferation capacity compared to those of healthy individuals [10–12]. Finally, the repetitive regeneration of DMD muscle could reduce the proliferation potential of the muscle satellite cells, again making the model design difficult [13, 14]. These DMD myoblast phenotypes are considered secondary effects of chronic inflammation and therefore may not well represent the actual primary pathogenesis. On the other hand, since the establishment of human iPS cells in 2007 [2], iPS cell-based disease models have become invaluable resources for the study of disease mechanisms and drug screening [15, 16]. Because of their unlimited proliferation potential, patient-derived human iPS cells have tremendous advantages in drug screening over patient-derived somatic cells such as myoblasts or fibroblasts. Myoblasts induced from patient-derived iPS cells allow us to investigate primary pathogenesis which is generated by the loss of dystrophin itself without the conflation of secondary effects due to chronic inflammation. Additionally, myogenic differentiation from iPS cells allows us to repeatedly produce a large number of intact and homogeneous myotubes from healthy subjects and DMD patients in vitro.
Just as it was possible to identify a drug target through the accurate understanding of pathology in a genetic disease such as a sialic acid for GNE myopathy [17], a detailed analysis of pathogenesis in other intractable myopathies is also warranted. The majority of intractable myopathies are genetic disorders, and there is a high expectation that their pathology can be reproduced using in vitro models drawing on genetic information contained in patient-derived iPS cells.
This chapter will discuss how iPS cells are being used in cell transplant therapy and drug discovery for myopathies as well as studying the pathology of the disease.
9.2 Approaches for Cell Therapy
9.2.1 Basic Studies of Cell Therapy Using Mouse ES/iPS Cells
Using mouse models, muscle regeneration therapies based on the induction of mouse pluripotent stem cells to differentiate into skeletal muscle progenitor cells are being developed for transplantation experiments. Various methods have been reported to induce mouse ES cell differentiation to skeletal muscle. Darabi et al. were the first to successfully treat muscular dystrophy in a mouse model [18]. They produced skeletal muscle progenitor cells with high efficiency by forcing the expression of an indispensable transcription factor in the formation of skeletal muscle, Pax3, in ES cells using a tetracycline induction system. However, when whole differentiated cells were transplanted into mice, teratomas, a major adverse effect of ES cell transplants, occurred due to mixing with undifferentiated cells. Using a DMD mouse model (mdx mice), they transplanted a purified cell fraction that included PDGFRa positive and Flk1 negative, which are markers for somite and paraxial mesoderm [19], which is the embryonic origin of skeletal muscle [20]. The transplanted cells fused to host myofibers and the muscle fibers expressed dystrophin which is encoded by Dmd, a causative gene of DMD. Furthermore, the contractile force of isolated skeletal muscle and motor function of treated mouse improved. Additionally, engraftment and regeneration were found not only with direct administration to the skeletal muscle but also via vascular administration. However, it was still unclear at that time whether the transplanted cells were engrafted as satellite cells or were just fused to host myofiber like myoblasts.
In the same year, I and colleagues induced the differentiation of mouse ES cells into mesoderm cells in 2D culture without forcing gene expression and found that the PDGFRa-positive fraction contained skeletal muscle progenitor cells [21]. In vivo differentiation into satellite cells was reported when these PDGFRa-positive cells were transplanted into mice with muscle damage. A portion of the transplanted, ES cell-derived cells expressed Pax7, which is a known molecular marker for satellite cells, and differentiated into multinucleated, mature muscle fibers when isolated from live mice and cultured, although the efficiency of the differentiation was low. Chang et al. induced the differentiation of mouse ES cells into mesoderm cells by embryoid body formation and succeeded in isolating cells with a high capacity for muscle regeneration [22] by using markers specific to resting-state satellite cells called SM/C-2.6 [23]. Dystrophin expression was restored by locally transplanting these cells into the skeletal muscle of mdx mice, and their differentiation into satellite cells was confirmed. Additionally, when the ES cell-derived satellite cells were isolated from skeletal muscle and transplanted again, they fused to skeletal muscle as expected, confirming their differentiation into satellite cells in vivo. This work is the first published report to show mouse ES cell-derived cells differentiated into functional satellite cells and to demonstrate repeated muscle regeneration in vivo. In 2011, Darabi et al. showed that mouse ES cells could be induced to become skeletal muscle progenitor cells through forced expression of not only Pax3 but also Pax7 [24]. In this report, the transplanted ES cell-derived cells differentiated into Pax7-positive cells in vivo and exhibited regenerative capacity, indicating that the Pax7-mediated ES cell-derived muscle progenitors were engrafted as functional satellite cells.
Following the development of mouse iPS cells [1], SM/C-2.6-positive cells were isolated from mouse iPS cells and found to engraft as satellite cells in vivo the same way as mouse ES cells [25]. Later, it was discovered that mouse iPS cells could also be induced to differentiate into skeletal muscle progenitor cells by the forced expression of Pax7 [26]. Moreover, mouse iPS cells were induced to differentiate into PDGFRa-positive/Flk1-negative cells without genetic recombination and found to engraft as satellite cells in vivo following transplantation [27]. Thus, the myogenic progenitor production found in mouse ES cells was reproduced in mouse iPS cells, demonstrating that mouse iPS cells are equivalent to mouse ES cells in their ability to differentiate into muscle cell lineages based on the induction of satellite cells.
9.2.2 Basic Studies of Cell Therapy Using Human ES/iPS Cells
Parallel to the mouse studies above, research on the induction of human pluripotent stem cells for differentiation into satellite cells is being conducted for clinical application in cell transplant therapies. These studies are expected to be especially effective, because human iPS cells can be obtained from the patients themselves, which reduces the immune rejection response. However, since muscular dystrophy is a genetic disease, patient cells cannot be used as a treatment strategy without gene repair. Although the dystrophin gene, the causative factor of DMD, is a very large gene, which makes complete repair difficult, Kazuki et al. succeeded in patient-derived iPS cells using artificial human chromosome techniques [28]. Due to this advancement, expectations for research in the development of muscle stem cell transplant therapies using iPS cells have grown.
The first induction of human ES cells to differentiate into myoblast cells was reported in 2007 [29]. In that paper, surface marker molecules such as CD73 and NCAM were used to isolate myoblast cells, which were obtained approximately 50 days after induction. Although these cells did not turn into satellite cells upon transplantation, they did show similar local regenerative capability. However, the differentiation potential varied tremendously between human pluripotent stem cell clones, including those established from the same donor [30]. Although a very interesting finding, because this method is difficult to reproduce, alternative induction protocols have been sought. Two include using either a TGF-beta signal inhibitor, SB-431542 [31], or retinoic acid [32]. However, while both promote the differentiation of skeletal muscle, neither could be used to induce differentiation into satellite cells nor to achieve successful engraftment. Subsequently, similar to mouse ES/iPS cells, it was reported that progenitor cells, including those derived from human iPS cells, with the ability to differentiate into muscle are present in the PDGFRa-positive/Flk1-negative population, although again, differentiation into satellite cells and engraftment in vivo was not observed [27]. Awaya et al. succeeded to induce differentiation into skeletal muscle progenitor cells using an improved culturing method and marker-based separation without performing forced gene expression [33]. They found that human iPS cell-derived muscle progenitors differentiated into Pax7-positive satellite cells in vivo when transplanted into cardiotoxin treated regeneration model muscle of immunocompromised mice.
Since then, many important reports on cell transplant therapy using muscular dystrophy mouse models have been published. Darabi et al. applied a method originally used to induce skeletal muscle progenitor cells from mouse ES/iPS cells on human ES/iPS cells and succeeded in obtaining skeletal muscle progenitor cells with high efficiency by forced Pax7 expression [34]. There, human ES/iPS cells were first differentiated into mesoderm cells using the embryoid body (EB) method, and then time-specific expression of Pax7 was forced using a tetracycline-inducible system. After purifying Pax7 positive cells, the cells were induced to become muscle progenitor cells at a purity of 99 % when cultured with continuous forced expression of Pax7. When these skeletal muscle progenitor cells were transplanted into immunocompromised mdx mice, human nucleus-positive/human dystrophin-positive muscle fibers were formed, and it was found that the transplanted cells participated in the muscle regeneration. Additionally, some of the transplanted cells engrafted into muscle fibers as Pax7-positive satellite cells, and as much as a year after transplantation, human/dystrophin-positive muscle fibers were confirmed, suggesting that the transplanted cells participated in the repair as satellite cells for long term in vivo. Unfortunately, despite these promising results, this method involved a lentivirus vector for recombination into the genome, which is considered unsafe for clinical purposes.
Similarly, Tedesco et al. reported successful muscle regeneration by inducing human iPS cells to differentiate into cells that had characteristics similar to fetal-stage vascular progenitor cells called mesoangioblasts and subsequently transplanting these cells into diseased muscles of limb-girdle muscular dystrophy 2D model mice [35]. They reported that human iPS cells could differentiate into mesoangioblast-like cells that were previously established from human periarterial cells [36] by changing the culture conditions at four different stages over a 1-month period. Though these iPS cell-derived mesoangioblast-like cells rarely produced skeletal muscle spontaneously in vitro, if the master gene for skeletal muscle differentiation, myogenic differentiation 1 (MyoD1), was forcibly expressed during transplantation into a mouse model, the cells could adhere to the mouse muscle fibers and participate in the muscle regeneration. Furthermore, engraftment in the skeletal muscle was verified not only by intramuscular injection but also by administration into the arteries, suggesting that the iPS cell-derived mesoangioblast-like cells are excellent progenitor cells capable of systemic administration into model animals. The same team also succeeded in inducing mesoangioblast-like cells in iPS cells derived from muscular dystrophy patients. However, differentiation into satellite cells in vivo was not obtained. Following that work, Goudenege et al. reported that by inducing differentiation of human ES/iPS cells into mesenchymal cells over approximately a week and then forcing the expression of MyoD1 using an adenovirus vector, skeletal muscle progenitor cells could be generated with an efficiency of approximately 40–60 % [37]. They showed that, through intramuscular injection, iPS cell-derived skeletal muscle progenitor cells could provide a similar level of muscle regeneration to myoblast cells. They also succeeded in inducing differentiation in iPS cells derived from muscular dystrophy patients. However, differentiation into satellite cells in vivo was again not obtained. Additionally, while adenovirus vectors offer the advantage of avoiding recombination with the genome, they are considerably immunogenic such that a different vector is desirable for clinical use. The above studies show that successful muscular regeneration following transplantation can be achieved by inducing muscle progenitor cells through the forced expression of Pax7 or MyoD1. More recently, however, Xu et al. induced the differentiation of engraftable muscle progenitor cells to skeletal muscle following transplantation without any gene modification by using a differentiation culture with additives including the respective bFGF and Wnt signal agonists Bio and forskolin [38].
These findings demonstrate the use of human iPS cell-derived cells for muscle regeneration in mouse dystrophy models. The discovery of markers such as Pax7 will be instrumental in verifying whether satellite cells can be induced from hiPSCs and whether posttransplant engrafted cell populations really function as satellite cells. Future work is also required to evaluate the efficacy and safety of these transplant therapies by analyzing the motor function of individual mice and expanding the long-term observations of these transplantations in animal models with long life-spans such as dog.
9.3 Approaches for Drug Screening Followed by Disease Modeling Using Patient-Derived iPS Cells
9.3.1 Establishment of Efficient and Reproducible Differentiation Methods from Human iPS Cells to Muscle Cells
Drug development research using patient-derived iPS cells requires a highly efficient and reproducible method for inducing the differentiation to the target tissue. However, even as research into skeletal muscle progresses, most reports to date about the induction of human ES/iPS cells for differentiation into skeletal muscle cells are poor in these metrics. In order to initiate skeletal muscle differentiation, we forcibly expressed the master transcription gene, MyoD1, in undifferentiated human iPS cells to achieve an efficiency approaching 90 % [39]. MyoD1 expression was controlled by using a tetracycline-responsive vector in an extremely simple method that involved only the addition of doxycycline to the culture medium. The reproducibility and efficiency of this method is extremely high even when carried out on various iPS cell lines including patient-derived iPS cells [39]. Moreover, the cells induced by this protocol showed promising phenotypes, including fusion with each other and contraction upon electrical stimuli, indicating mature muscle cells [39]. Through comprehensive gene expression analysis and structural analysis using electron microscopy, we showed that these iPS cell-derived muscle cells exhibited similarities to primary myoblasts obtained through human biopsies [39]. Utilizing the above results, we carried out studies to reproduce pathologies in muscle cells differentiated from patient-derived iPS cells, as described below.
9.3.2 Modeling Muscular Dystrophy Using Patient-Derived iPS Cells
9.3.2.1 Modeling Miyoshi Muscular Dystrophy
Miyoshi myopathy is an autosomal recessive, hereditary distal myopathy that was first reported by Miyoshi et al. in 1986 [40]. The disease may present itself from the age of 10 years and causes difficulty in walking or standing on the toes. It is a progressive disease with gradual muscle loss, and the causative gene was identified as the dysferlin gene [41]. It was confirmed that dysferlin functions to repair muscle cell membranes [42], and it is believed that the muscle cells become damaged due to delayed membrane repair, which leads to the onset of the myopathy [43]. However, the mechanism by which the delayed membrane repair causes the degeneration and death of muscle cells is not well understood. We aimed to reproduce this abnormal muscle cell membrane repair pathology using iPS cells derived from Miyoshi myopathy patients. Adopting the forced expression of MyoD1 described above, we induced mature muscle cells from Miyoshi myopathy patient-derived iPS cells with high efficiency [39]. Compared to healthy control cells, there was no difference in the induction efficiency, rate of differentiation, or marker molecule expression in the cells obtained. Next, to reproduce the muscle repair pathology, a membrane damage experiment was performed using two-photon laser confocal microscopy, whereby the differentiated muscle cells were perforated by laser irradiation. The time required for membrane repair was calculated by measuring the time taken for a fluorescent dye added to the culture medium to be taken into the cells. Because repair of the muscle cell membrane occurs on a timescale of a few seconds for healthy control iPS cell-derived muscle cells, we found that these cells did not increase their uptake of fluorescent compounds after 1 min. However, for muscle cells from Miyoshi myopathy patient-derived iPS cells, the intake of fluorescent compounds continued even after 5 min [39]. As mentioned earlier, clones of human iPS cells show a large variation in differentiation potential [30]. To verify that the differences between healthy control cells and patient-derived cells were not due to the variation between clones, gene-repaired, new patient-derived iPS cells were produced whereby dysferlin was restored by the forced expression of dysferlin cDNA. These cells were made to differentiate into skeletal muscle cells, and, on carrying out similar membrane damage experiments, it was found that the prolonged fluorescent compound uptake was shortened compared to that before the restoration [39]. In other words, we successfully reproduced the pathology of the lengthened membrane repair time and found that it was clearly due to dysferlin deficiency.
Based on these results, we are developing new methods of a membrane damage-inducing system which can be adopted for multi-well plate-based drug screening. It is expected that in the near future, drug screening using muscle cells from patient-derived iPS cells to augment muscle cell membrane repair will be possible.
9.3.2.2 Modeling Duchenne Muscular Dystrophy
DMD is a severely debilitating and intractable disease that occurs in 1 of every 3,500 male children born [44]. It is caused by damage or mutation of the dystrophin gene on the X chromosome [45], which leads to muscle degeneration caused by progressive inflammation and necrosis of the skeletal muscle. The disease has an exceptionally poor prognosis, with management using artificial respirators by the time the patient is in his 20s and death in his 30s. At present, there is no curative treatment, with steroids being the only drug used to slow progression [46]. Collaborative international clinical trials using antisense oligonucleotides for exon skipping in DMD are underway [47]; however, only limited number of DMD patients who have specific exon deletions can be applied by this treatment. Thus, a drug treatment that shows positive effects in all DMD patients is preferred. However, more than a quarter century since the identification of dystrophin [45], the question of why dystrophin deficiency causes serious, chronic inflammation in skeletal muscle remains unanswered.
Continued research using the DMD mouse model, mdx, has suggested an excess flow of Ca2+ within muscle cells as a likely cause [48]. This hypothesis argues that if a state of high intracellular Ca2+ concentration continues unabated, not only do the mitochondria become damaged [49], which increases oxidative stress, but abnormal activation of the NF-kB pathway leads to chronic inflammation [50]. Indeed, when genetic modifications were made to restrain the activity of a stretch-activated ion channel, transient receptor potential vanilloid 2 (TRPV2), in mdx mice, a reduction in muscle tissue inflammation was reported [51]. In contrast, in healthy mice expressing dystrophin, overexpression of another stretch-activated ion channel, transient receptor potential canonical 6 (TRPC6), reproduced the pathology of muscular dystrophy [52].
On the suspicion that this excess in intracellular Ca2+ flow triggers the DMD pathology, we undertook recapitulating the pathology in DMD patient-derived iPS cells (DMD-iPSCs). Using electrical stimuli, we contracted these differentiated skeletal muscle cells, and, using Ca2+ imaging and a fluorescent probe, we measured the Ca2+ concentrations during the contraction [53]. DMD-iPSC derived myocytes demonstrated higher Ca2+ influx than control iPS-derived myocytes [53]. Furthermore, when dystrophin expression was restored by exon-skipping in DMD-iPSCs, excess Ca2+ influx was attenuated [53]. Based on these results, we successfully demonstrated that excessive Ca2+ influx is one of the earliest events to occur in dystrophin-deficient muscle during contraction [53]. We have been investigating to apply our DMD modeling system as a screening method for drugs that target the excess Ca2+ flow.
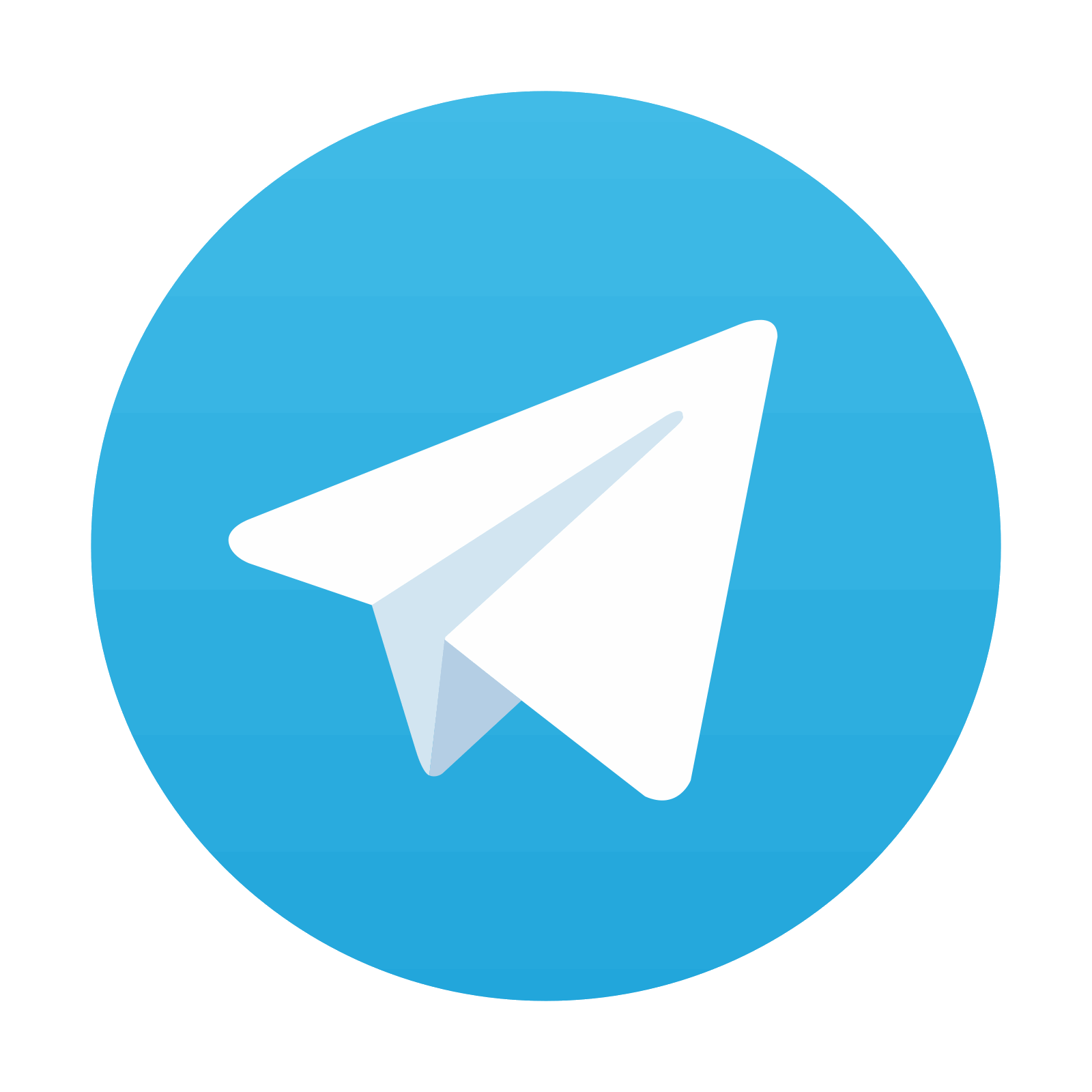
Stay updated, free articles. Join our Telegram channel
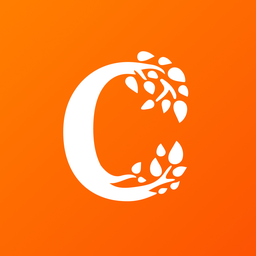
Full access? Get Clinical Tree
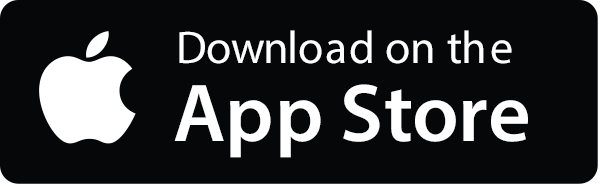
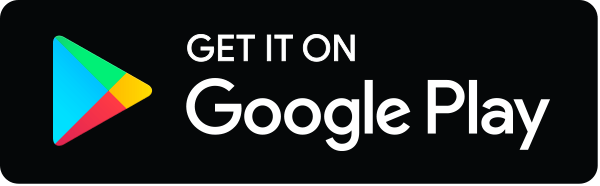
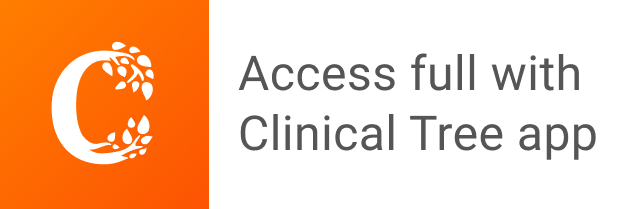