Fig. 8.1
Transcriptional and translational control of skeletal muscle PGC-1α. Exercise is a potent regulator of PGC-1α in muscle. Increases in calcium concentration (Ca2+) activate the p-38 mitogen-activated protein kinase (MAPK) pathway. This indirectly enhances PGC-1α gene expression via the phosphorylation (P) of the activating transcription factor-2 (ATF-2) that binds to PGC-1α promoter. Additionally, PGC-1α gene expression is increased by the exercise-induced attenuation of promoter methylation (Meth). miRNA binding to the 3′ untranslated region of the PGC-1α gene can result in mRNA degradation or inhibit protein translation. Post-translational regulation of PGC-1α activity can occur via the exercise-induced depletion of ATP and consequent activation of 5′ AMP-activated protein kinase (AMPK), which can directly phosphorylate and activate PGC-1α. PGC-1α is also targeted by other exercise-activated kinases, including protein kinase B (PKB, also known as Akt), ribosomal protein S6 kinase beta-1 (S6K1, also known as p70-S6K) and glycogen synthase kinase 3 beta (GSK3β). Further post-translational modifications include acetylation, deacetylation, ubiquitination and methylation
8.3 microRNA Regulation of PGC-1α
microRNAs (miRNAs) are small non-coding RNAs that play a major role in skeletal development and adaptation to various physiological and pathophysiological situations [67, 96]. miRNAs inhibit protein expression [38, 56] either by degrading specific mRNA species or by repressing their protein translation. The miRNA inhibitory activity most commonly occurs through the binding of the 5′ end of the miRNA with the 3′ UTR of the target mRNA, although miRNA targeting the 5′UTR may also occur. Overexpression of miR-696 in C2C12 myotubes negatively regulates PGC-1α protein with a corresponding decrease in two PGC-1α-regulated genes, pyruvate dehydrogenase kinase 4 (PDK4) and cytochrome c oxidase subunit 2 (COXII) as well as fatty oxidation [2]. However, it was not established if miR-696 directly regulates PGC-1α via 3′ UTR binding.
miR-23a binds directly to the PGC-1α 3′ UTR in C2C12 myotubes, repressing its mRNA levels, while transgenic mice overexpressing miR-23a have a reduction in PGC-1α, cytochrome b and cytochrome c oxidase complex IV (COX IV) protein levels [68]. In miR-23a transgenic mice, when compared with wild-type mice, protein markers of mitochondrial content, including PGC-1α and COX IV, are significantly decreased in the slow soleus muscle, but not the fast plantaris muscle [85]. However, the miR-23a reduction in PGC-1α did not affect the adaptation to 4 weeks of voluntary wheel running, with increases in endurance exercise capacity, muscle mass, capillary density and the protein content of myosin heavy chain IIa, PGC-1α, COX IV and cytochrome c observed. It appears that miR-23a targets PGC-1α and regulates basal metabolic properties of slow but not fast-twitch muscles, but does not influence whole-body endurance capacity or exercise-induced muscle adaptations in the fast plantaris muscle [85].
8.4 Roles of the PGC-1 Family Members in Skeletal Muscle
Skeletal muscle has a rare capacity to continually adapt to stress exerted by its external environment. With such a high level of expression and rapid regulation in skeletal muscle, PGC-1α and PGC-1β are important players in numerous adaptive processes. These include mitochondrial biogenesis, muscle fibre type, substrate metabolism, angiogenesis, inflammation and neuromuscular junction formation (Fig. 8.2). The majority of our understanding of the potential role of PGC-1α and PGC-1β has come from transgenic gain and loss-of-function mouse models. However, the physiological impact of exogenous PGC-1α and PGC-1β overexpression appears to be influenced by the promoters used, the tissues affected and the intensity of the transgenic regulation. Therefore, a considered approach should be taken when interpreting certain findings based on transgenic PGC-1α and PGC-1β models, in relation to the potential role of endogenous PGC-1α and PGC-1β.


Fig. 8.2
Various processes regulated by PGC-1α and/or PGC-1β in skeletal muscle
8.5 Mitochondrial Biogenesis
In vitro, the overexpression of PGC-1α [94] or PGC-1β [77] in myotubes increases mitochondrial mass. In mice with a muscle-specific transgenic overexpression of PGC-1α [44, 90] or PGC-1β [5], there is an increase in genes involved in mitochondrial biogenesis, function and oxidative metabolism. In skeletal muscle cells and tissue, PGC-1α and PGC-1β exert their positive effect on mitochondrial biogenesis through its direct interaction and coactivation of ERR and NRF family members [22, 53, 73, 75]. It appears that PGC-1α is not required for basal mitochondrial biogenesis as PGC-1α knockout mice have a very mild attenuation in mitochondrial function [27, 41]. In contrast to PGC-1α knockout mice, the ablation of PGC-1β, specifically in mouse skeletal muscle, decreases in expression of genes controlling mitochondrial protein import, translational machinery and energy metabolism, leading to impaired mitochondrial structure and function [24].
8.6 Muscle Fibre Type
Transgenic overexpression of PGC-1α in fast type IIb glycolytic muscles results in a conversion to the more oxidative slow-twitch type I and IIa fibres [44]. PGC-1β increases the fast-oxidative type IIX muscle fibres via its coactivation of the MEF2 transcription factor family [5]. The high oxidative capacity of these muscle fibres is due to their enrichment in the mitochondria that is influenced by the PGC-1α and PGC-1β coactivation of ERRα and NRF1. However, as PGC-1α and PGC-1β knockout mice appear to have a normal muscle fibre composition, other factors also play a major role in fibre-type determination [4, 24].
8.7 Substrate Metabolism
Both PGC-1α and PGC-1β regulate the expression of many genes involved in energy metabolism and substrate oxidation [30], and many of these effects are dependent on ERRα [10, 73, 89]. PGC-1α or PGC-1β overexpression in rodent skeletal muscle increases glucose transporter type 4 (GLUT4) levels as well as insulin-stimulated glucose transport [8, 36]. Their overexpression also increases the expression of numerous genes involved in fat oxidation, such as the fatty acid transporter, CD36 [8, 33, 81]. PGC-1α or PGC-1β overexpression in L6 myotubes also increases creatine transporter mRNA levels and creatine uptake via ERRα [10].
8.8 Angiogenesis
Increase in skeletal muscle capillary density, a process referred to angiogenesis, is an important adaptation to exercise and plays a role in improved endurance performance. This allows for increased blood flow and nutrients to enter the muscle. PGC-1α and PGC-1β, through hypoxia-inducible factor (HIF)-independent but oestrogen-related receptor-α (ERRα)-dependent activation, increase the gene expression of vascular endothelial growth factor (VEGF), a key player in angiogenesis [3, 63]. PGC-1α also positively influences angiogenesis via its upregulation of secreted phosphoprotein 1 (SSP1) [64]. This PGC-1α/SSP1 regulatory axis stimulates macrophage activation and recruitment. As observed in many other situations, it appears that PGC-1α [16] is not required for basal angiogenesis. However, it is required for exercise-induced angiogenesis [40].
8.9 Neuromuscular Junction Formation
The neuromuscular junction (NMJ) is a synapse that forms a connection between the motor neuron and the muscle fibres. In doing so the NMJ mediates signals that are important for muscle activity. PGC-1α overexpression positively regulates NMJ formation and stability [6, 29]. Ablation of PGC-1α reduces gene expression of a number of NMJ-related genes such as acetylcholine receptor (AChR) and utrophin [29]. As such the manipulation of PGC-1α levels has been considered as a potential therapeutic approach for diseases with impaired NMJ function such as Duchenne muscular dystrophy (DMD) and amyotrophic lateral sclerosis (ALS). In the mdx mouse model of human DMD, transgenic overexpression of skeletal muscle PGC-1α improves muscle histology, running performance and plasma creatine kinase levels [29]. Similarly, viral-induced overexpression of PGC-1α in neonatal mdx mice increased utrophin and type I myosin heavy chain expression. There was also an increase in mitochondrial protein content as well as resistance to contraction-induced damage and fatigue [74]. Viral-induced overexpression of PGC-1α in 1-year-old mdx mice increases muscle tension and force production, when measured 3 months postinjection. However, histopathology was not improved [31]. Overexpressing PGC-1β in skeletal muscle of mdx mice also improves muscle function, without significantly impacting the NMJ [14]. Skeletal muscle overexpression of PGC-1α in fast-twitch muscles of SOD1G37R ALS mouse model improves skeletal muscle function and mitochondrial biogenesis, but does not preserve neurodegeneration or extend survival [18].
8.10 Inflammation
In multiple conditions of disuse and inactivity, as well as in disease conditions, systemic inflammation is often observed [23, 49], a response commonly associated with reduced levels of skeletal muscle PGC-1α and PGC-1β [50, 58, 68]. Often the inflammatory response is stimulated by a discordant metabolic and immune response resulting in the accumulation of pro-inflammatory macrophages that release pro-inflammatory cytokines such as tumour necrosis factor alpha (TNF-a), interlukin-6 (IL-6) and monocyte chemotactic protein 1 (MCP-1) [55]. Skeletal muscle ablation of PGC-1α results in an increase in both local skeletal muscle and systemic inflammation [28]. In C2C12 muscle cells, the overexpression of PGC-1α and PGC-1β attenuates the upregulation of pro-inflammatory cytokine expression following treatment with TNFα, a response mediated via the PGC-1 reduction in p65 phosphorylation (NF-κB family member) [21]. In vivo, mice with a skeletal muscle transgenic overexpression of either PGC-1α or PGC-1β have an altered anti-inflammatory profile characterized by increases in C-C motif chemokine ligand 1 (CCL1), CCL22, interleukin 1 receptor antagonist (IL-1Ra), transforming growth factor β (TGFβ) or interlukin-10 (IL-10). This was also associated with a decrease in interlukin-12 (IL-12) [20].
8.11 Expression of the PGC-1 Family Members in Models of Skeletal Muscle Use, Disuse and Disease
The multifactorial role played by PGC-1α and PGC-1β in skeletal muscle makes them susceptible to high levels of regulation as the metabolic environment of the muscle changes. One important aspect of the plasticity of skeletal muscle is its ability to rapidly increase or decrease its mass in response to anabolic and catabolic stimuli. Expression of the PGC-1 proteins is reduced in conditions associated with skeletal muscle atrophy and perturbed metabolic function. Normal ageing is typically associated with a decrease in muscle mass and function that is exacerbated by physical inactivity [84]. PGC-1α and PGC-1β mRNA levels were higher in young (28 ± 0.2 years) than in elderly healthy adults (62.4 ± 0.2 years) [46], and muscle PGC-1α protein content was decreased in sedentary old adults (63 ± 3.2 years) when compared to active old adults (70 ± 1.6 years) and to young adults (22 ± 0.6 years) [70]. Immobilization, a model of muscle disuse, partially mimics the effects of age-related muscle wasting and induces accelerated muscle atrophy. In both young (21–30 years) and older (60–72 years) subjects, immobilization decreased PGC-1α and PGC-1β gene expression after 2 days and 4 days, while a decrease in PGC-1α mRNA was still visible after 14 days of muscle disuse [78]. Similarly, 5 days of one-legged knee immobilization decreased PGC-1α mRNA expression in healthy young (22 ± 1 years) subjects [86]. Muscle atrophy is also a secondary outcome of numerous metabolic or genetic disorders, which are frequently associated with attenuation in the activity and expression levels of the PGC-1 family members. When compared to healthy controls, PGC-1α and PGC-1β gene levels were reduced in muscle from patients with Huntington’s disease [15], a disease characterized by impaired oxidative phosphorylation [17], and from patients with type II diabetes [54, 58]. A collective attenuation of PGC-1 signalling genes is observed in the muscle of patients with amyotrophic lateral sclerosis (ALS). In addition to a decrease in PGC-1α and PGC-1β protein levels, their downstream targets ERRα, NRF1, MFN1, MFN2 and COX IV were downregulated, reflecting a profound disruption of the whole mitochondrial network [68]. Finally, comparable findings have been reported in animal models. PGC-1α protein levels were notably reduced in a rat model of congestive heart failure [82], while PGC-1α gene levels were attenuated in rats displaying cancer cachexia, chronic renal failure or submitted to denervation [69, 72]. Overexpressing PGC-1α in cultured muscle fibres provided an insight into the molecular mechanisms underlying its role in muscle atrophy. It was proposed that PGC-1α protects muscle mass by attenuating the transcriptional activity of FOXO3a, a central activator of muscle protein degradation [9, 72]. In line with this hypothesis, overexpressing PGC-1α in rodent muscle limited muscle atrophy induced by age [90] and immobilization [35].
Endurance exercise is a potent activator of mitochondrial biogenesis and oxidative metabolism in muscle [32]. As such, a single bout of exercise induces the PGC-1 family members in skeletal muscle, with little distinction between exercise types and regimes. An early study showed that PGC-1α mRNA increased at 2 and 6 h following 3 h of two-legged knee extensor exercise [60]. Ten kilometre cycling time trial induced mRNA expression of PGC-1α and its coactivator ERRα 2 hours postexercise while activating the mitochondrial regulators Mfn1, Mfn2, NRF-2 and COX IV 24 hours postexercise [13]. Ninety minutes interval and continuous cycling exercise at a similar intensity equally increased PGC-1α mRNA [88]. Interestingly, resistance exercise also has the ability to induce the PGC-1 family members. Following a single session of endurance or resistance exercise, there was an increase in PGC-1α mRNA 30 min and 180 min after exercise, respectively, with no change in PGC-1β [76]. Finally, resistance exercise potentiated the endurance-induced activation of PGC-1α by increasing its mRNA levels by twofold more than following endurance exercise alone [87].
Exercise training stimulates the expression of the PGC-1 family, suggesting a sustained role of the PGC-1 proteins in muscle adaptation to exercise and remodelling. PGC-1α mRNA expression increased in healthy men undergoing 6 weeks of endurance training, with a greater relative increase observed in type IIa fibres than in type I and IIx fibres [66]. Eight weeks of either endurance or resistance training independently enhanced PGC-1α mRNA expression, but as it is the case for a single bout of exercise, combined training regimes potentiated this activation [65]. While PGC-1α mRNA expression consistently increases with endurance training, one study reported an increase in mitochondrial function that was not associated to an increase in PGC-1α protein expression in response to 2 weeks of high-intensity interval training (HIT) [83], while 6 weeks of HIT increased PGC-1α protein content by 100 % [11]. Such discrepancies may result from differences in PGC-1α protein subcellular localization. At rest, PGC-1α is localized in the cytosol, but its presence in the nucleus increases following endurance exercise [71, 92]. There is also evidence suggesting that PGC-1α can shuttle to the mitochondria to allow Tfam coactivation [71]. As a result, total PGC-1α protein content may not always be reflective of PGC-1α activation [92].
While the roles of the PGC-1 family members in suppressing protein degradation and enhancing mitochondrial metabolism have been independently established, it is less clear whether the PGC-1 proteins have the capacity to trigger muscle hypertrophy by enhancing muscle protein synthesis. PGC-1α did not transiently increase protein synthesis in C2C12 myoblasts [9], and one study showed that the hypertrophy response induced by chronic overload of skeletal muscle was independent of PGC-1α [59]. A recently described truncated spliced variant of PGC-1α, PGC-1α4, was expressed in resistance-trained mice and humans [65]. In humans, 8 weeks of resistance training increased PGC-1α4 expression, and its expression levels were correlated with leg press exercise performance. Rather than regulating genes involved in cell bioenergetics, PGC-1α4 stimulated muscle hypertrophy in vitro and in mice by inducing insulin growth factor-1 (IGF-1), the primary stimulator of the PI3/AKT pathway, one of the major protein synthesis signalling routes in muscle [62]. It also repressed the myostatin pathway, an inhibitor of AKT and negative regulator of muscle mass [79]. Induction of PGC-1α4 was however not resistance exercise specific [47, 95], and exercise-induced PGC-1α4 expression did not correlate with changes in muscle size or muscle strength, questioning the role for PGC-1α4 in human muscle hypertrophy.
8.12 Conclusion
Since the discovery of the PGC-1 family, a considerable amount of work has been performed to identify molecules that activate and inhibit its function, as well as the downstream transcription factors that they coactivate. The development of several gain- and loss-of-function mouse models has identified the pleiotropic roles that the PGC-1 family plays, especially in skeletal muscle. This has established the PGC-1 proteins as potential targets to treat diseases characterized by metabolic and neuromuscular impairments, as well as conditions such as ageing and immobilization. As the PGC-1 family are expressed in numerous tissues and are involved in the physiological adaptation to multiple stress situations, their endogenous levels are often downregulated in many pathological conditions. However, a causal role for attenuated PGC-1 activity and disease remains equivocal. Presently the development of pharmacological interventions to regulate PGC-1 family members has eluded the field and remains a research priority into the future. With this in mind, caution is required as excessive upregulation of PGC-1α can cause cardiomyopathy [39], skeletal muscle atrophy [52] and neurodegeneration [19]. Therefore, the ability to modulate the amount of PGC-1 activity in specific tissues will also present a challenge to the field.
References
1.
Andersson U, Scarpulla RC (2001) Pgc-1-related coactivator, a novel, serum-inducible coactivator of nuclear respiratory factor 1-dependent transcription in mammalian cells. Mol Cell Biol 21:3738–3749CrossRefPubMedPubMedCentral
2.
3.
4.
Arany Z, He H, Lin J, Hoyer K, Handschin C, Toka O, Ahmad F, Matsui T, Chin S, Wu PH, Rybkin II, Shelton JM, Manieri M, Cinti S, Schoen FJ, Bassel-Duby R, Rosenzweig A, Ingwall JS, Spiegelman BM (2005) Transcriptional coactivator PGC-1 alpha controls the energy state and contractile function of cardiac muscle. Cell Metab 1:259–271CrossRefPubMed
5.
6.
Arnold AS, Gill J, Christe M, Ruiz R, McGuirk S, St-Pierre J, Tabares L, Handschin C (2014) Morphological and functional remodelling of the neuromuscular junction by skeletal muscle PGC-1alpha. Nat Commun 5:3569CrossRefPubMedPubMedCentral
7.
8.
Benton CR, Nickerson JG, Lally J, Han XX, Holloway GP, Glatz JF, Luiken JJ, Graham TE, Heikkila JJ, Bonen A (2008) Modest PGC-1alpha overexpression in muscle in vivo is sufficient to increase insulin sensitivity and palmitate oxidation in subsarcolemmal, not intermyofibrillar, mitochondria. J Biol Chem 283:4228–4240CrossRefPubMed
9.
Brault JJ, Jespersen JG, Goldberg AL (2010) Peroxisome proliferator-activated receptor gamma coactivator 1alpha or 1beta overexpression inhibits muscle protein degradation, induction of ubiquitin ligases, and disuse atrophy. J Biol Chem 285:19460–19471CrossRefPubMedPubMedCentral
10.
11.
Burgomaster KA, Howarth KR, Phillips SM, Rakobowchuk M, Macdonald MJ, McGee SL, Gibala MJ (2008) Similar metabolic adaptations during exercise after low volume sprint interval and traditional endurance training in humans. J Physiol 586:151–160CrossRefPubMed
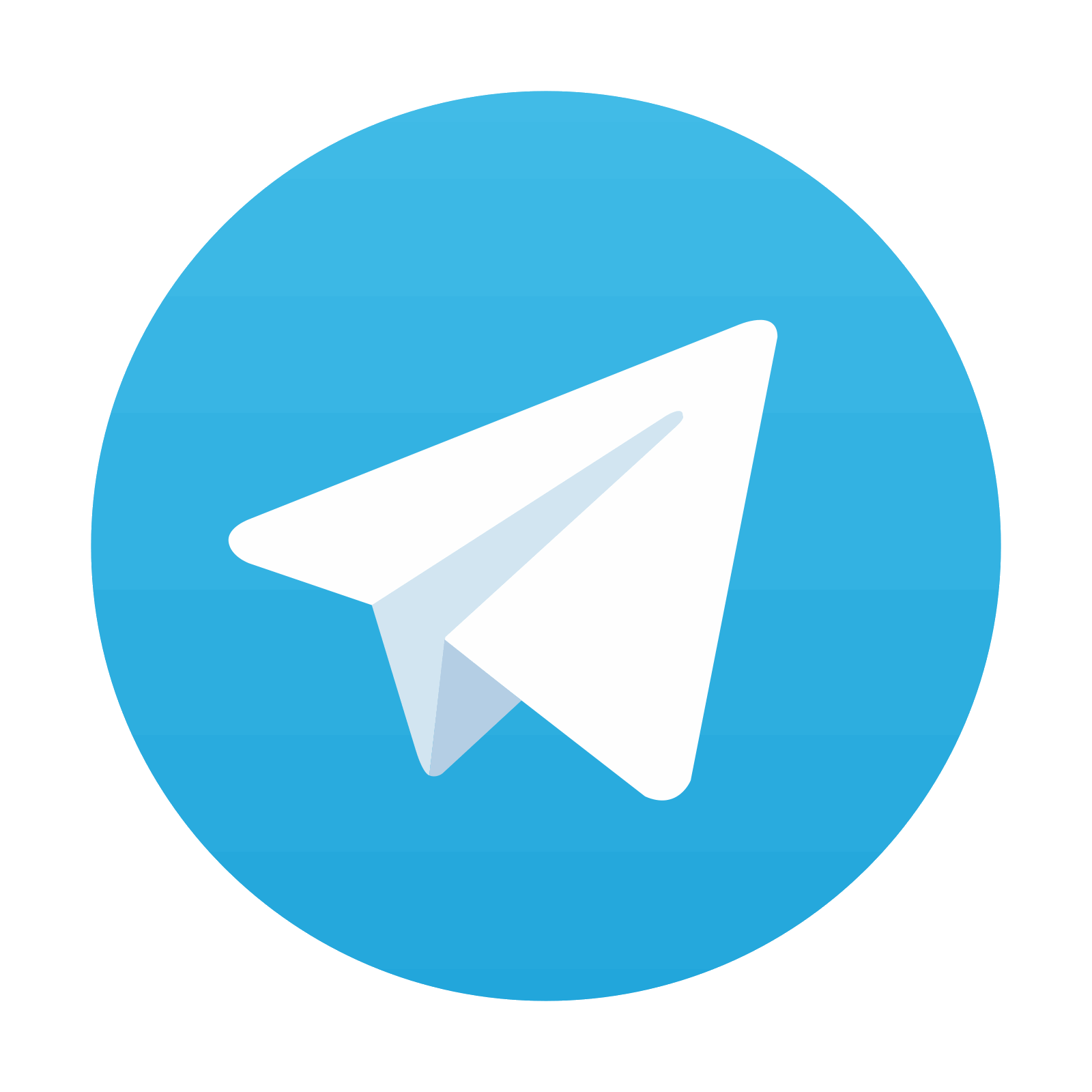
Stay updated, free articles. Join our Telegram channel
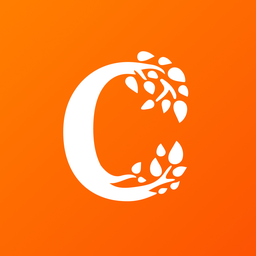
Full access? Get Clinical Tree
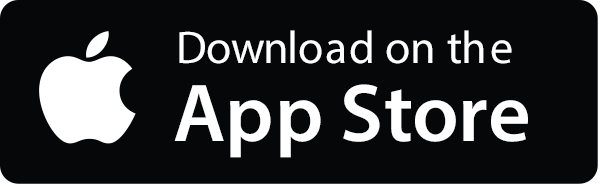
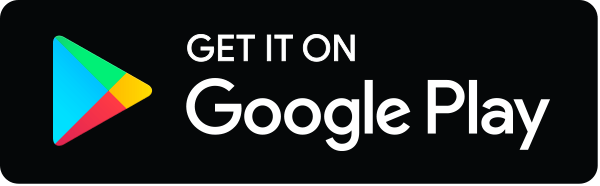