1
SEX HORMONES
Mary Caldwell, Ellen Casey, Bethany Powell, and Sandra J. Shultz
INTRODUCTION
Hormones affect the entire neuromusculoskeletal system in various ways depending on age, sex, and hormone concentration. Hormonal changes, which can be seen in the pubertal or the aging athlete, as well as during the menstrual cycle, may influence sports-related injury or sports performance. Therefore, the clinician treating musculoskeletal conditions must understand the implications of these hormonal changes in the male versus female athlete. In order to best approach this topic, we begin with a discussion of the key hormones in sexual development as well as their production and concentration across the life span. We then focus on the individual and combined hormonal effects on the many tissues in the musculoskeletal system, including a brief basic science review of collagen metabolism and function. We combine the findings from clinical studies and animal models to explore how hormonal differences between males and females may have implications on sports performance and sports-related injury. We end with a review of what we know about the influence of hormonal contraceptives on the musculoskeletal system and the role of sex hormones in anterior cruciate ligament (ACL) injury to highlight the clinical relevance of this material.
REVIEW OF HORMONAL PRODUCTION
The hormones produced by the human body are generally divided into four classes: steroids, peptides, amino acids, and fatty acids. Steroid hormones, such as estrogen, progesterone, and testosterone, are all fat-soluble molecules made from cholesterol. Growth hormone (GH), relaxin (1), and insulin-like growth factor 1 (IGF-1) are all water-soluble polypeptide hormones (2). This chapter focuses primarily on the steroid hormones and polypeptide hormones as they share the responsibility for growth and biologic dimorphism (3–5).
Steroid Hormones: Estrogen, Progesterone, and Testosterone
Progesterone, estrogen, and testosterone are created through the process of steroidogenesis (6–8). Steroidogenesis can be considered a single process that is repeated in various glands, such as the skin, ovary, or testicle, with cell-type specific variations (9). Cholesterol is converted to pregnenolone and then the specific hormone produced depends on the tissue and enzymes present (9). A steroid hormone is classified by the receptor it binds. Hence, while androgens, progestogens, and estrogens are considered steroid hormones, they are all of a different subclass since they bind to different steroid hormone receptors (10). Understanding the conversion and production of each of these hormones with relation to one another is crucial to interpreting the clinical relevance of hormonal imbalances with regard to biologic dimorphism and implications in sports medicine (Figure 1.1).
FIGURE 1.1: Steroidogenesis.
Estrogen is produced from the conversion of testosterone or androstenedione via the enzyme aromatase (11). In both males and females, production and conversion occur in adipose tissue, skin, muscle (8, ch. 13;12), and brain (13). In males, 80% of estrogen is produced by the peripheral glands while 20% is produced by the leydig cells in the testes (8, ch. 16). In premenopausal women, 95% of estrogen production occurs in the granulosa cells of the developing follicle during the proliferative phase and the corpus luteum during the luteal phase (LP) of the menstrual cycle (8, ch. 13). The most common estrogens are estradiol, estrone, and estriol. Estradiol is created primarily in the ovary and is the major form of circulating estrogen in nonpregnant females of reproductive age (8, ch. 13). Estrone, also primarily produced in the ovary, is the dominant type of estrogen present in postmenopausal women and has about one third of the potency of estradiol (8, ch. 13). Estriol is produced mainly in the placenta in pregnant women due to enzymes produced by the fetus and is biologically relevant only during pregnancy (8, ch. 13). Unless otherwise noted, estradiol is the form of estrogen discussed in this chapter. Estrogen acts primarily as an endocrine factor, but can also act as a paracrine factor. In the blood, 60% of estrogen is bound to albumin, 38% is bound to sex hormone-binding globulin (SHBG), and the remaining 2% is free (8, ch. 13) (Figure 1.2).
Progesterone is primarily produced by steroidogenesis in the corpus luteum during the LP of the menstrual cycle (8, ch. 13). During this phase, the granulosa cells convert thecal androgens to progesterone. The enzymes in the corpus luteum differ from the enzymes of the developing follicle, making progesterone synthesis favorable (8, ch. 13). In males, the leydig cells produce progesterone (8, ch. 12). Progesterone is also produced in the adrenal glands and nervous system by both sexes (6,7). Once progesterone enters the blood, 80% binds to albumin, 18% binds to corticosteroid-binding globulin, and 2% remains free (14) (Figure 1.2).
Testosterone is produced from steroidogenesis in the testes, ovaries, and adrenal glands. The testicular leydig cells in males produce over 95% of male testosterone, while in females, the ovaries produce the majority of testosterone. The adrenal glands also produce androgens called dehydroepiandrosterone (DHEA) and DHEA sulfate (DHEA-S) that are later converted to testosterone in multiple tissues, including the skin and muscle (12,15,16). In males, a small amount of testosterone is further converted to a five times more potent androgen called dihydrotestosterone (DHT) (17). Recall that in both sexes, testosterone can be converted to estrogen via the enzyme aromatase (11) (Figure 1.3).
Polypeptide Hormones: Relaxin, Growth Hormone, and Insulin-Like Growth Factor 1
Relaxin can be divided into two common types in humans: H2 and H3. H2 relaxin mainly binds the relaxin family peptide (RFXP) 1 receptor and is produced primarily by the corpus luteum and placenta (18). This isoform is also produced by the heart, blood vessels, breast, skin, liver, bone, and prostate (18). H3 relaxin is produced by the brain and testes and binds RXFP3 and RFXP1, although it binds RFXP1 with lower affinity than H2 relaxin (18). Relaxin, in this chapter, refers to H2 relaxin unless otherwise noted (Figure 1.4).
GH is produced by the pituitary gland, which then stimulates production and release of IGF-1 from the liver (5). IGF-1 mediates the anabolic actions of GH (19). The relationship between GH and IGF-1 is referred to as the GH/IGF-1 axis (20). Besides liver production, osteocytes can also secrete IGF-1 in response to mechanical loading (3) (Figure 1.5).
HORMONAL REGULATION
The neuro-endocrine axis regulates the production of hormones throughout the life span, causing levels to vary with age and sex. The hypothalamus releases gonadotropin-releasing hormone (GnRH) (8, ch. 15), growth hormone-releasing hormone (GHRH), and growth hormone-inhibiting hormone (GHIH), which act on the anterior pituitary (21). GnRH regulates the release of luteinizing hormone (LH) and follicle stimulating hormone (FSH) from the anterior pituitary gland (8, ch. 15), while GHRH and GHIH regulate the release of GH from the anterior pituitary gland (21) (Figures 1.2–1.5). GH then stimulates production and release of IGF-1 from the liver (5). Meanwhile, LH and FSH govern the production of estrogen, progesterone, and testosterone. Also, important in the regulation of estrogen and testosterone is sex hormone-binding globulin (SHBG). SHBG is produced primarily in the liver, but it is also produced in the uterus, brain, and testes. It essentially manages the bioavailability of the two main sex hormones (testosterone and estrogen). In general, SHBG decreases with high concentrations of androgens and increases with low concentrations of androgens (22). For example, if androgen levels are high, SHBG production is decreased proportionally, leading to appropriate activity of androgens. However, if there is too little SHBG for whatever reason, there can be excess androgen effects such as increased body hair and acne. Likewise, when androgen levels are low in response to the hypothalamic-pituitary-gonadal axis, SHBG production is increased to make a portion of the androgens biologically inactive (23,24). In contrast, SHBG levels increase with high-estrogen states such as pregnancy (22).
HORMONAL DIFFERENCES BY AGE, SEX, AND MENSTRUAL CYCLE
This section will discuss sex differences in hormonal concentrations throughout the life span. Please refer to Figure 1.6 for hormonal concentrations during the perinatal, prepuberty, puberty, male postpuberty, and female postmenopause time periods. Please refer to Figure 1.7 for hormonal concentrations during the female menstrual cycle.
FIGURE 1.2: Estrogen and progesterone in the neuromusculoskeletal system.
ACTH, adrenocorticotropic hormone; ACL, anterior cruciate ligament, CRH, corticotropic releasing hormone; FSH, follicle stimulating hormone; GnRH, gonadotropin releasing hormone; LH, luteinizing hormone.
FIGURE 1.3: Testosterone in the neuromusculoskeletal system.
ACTH, adrenocorticotropic hormone; FSH/LH, follicle stimulating hormone/luteinizing hormone; GnRH, gonadotropin releasing hormone.
FIGURE 1.4: Relaxin in the neuromusculoskeletal system.
FIGURE 1.5: Growth hormone and IGF-1 in the neuromusculoskeletal system.
GH, growth hormone; IGF-1/ILGF-1, insulin like growth factor 1.
FIGURE 1.6: Sex differences in hormonal concentrations throughout the life span. This figure is derived from multiple sources in order to compile a master life span perspective. Estrogen values for the perinatal period are estimated from work by Thompson et al. (25) while other time points are estimated from the NIH Clinical Center Lab references (cclnprod.cc.nih.gov/dlm/testguide.nsf/Index?OpenForm). The data points for testosterone and SHGB are derived from Elmlinger et al. (26). The data points for progesterone and IGF-1 are estimated from NIH Clinical Center Lab. Testosterone and SHGB are derived from Elmlinger et al. (26).
SHBG, sex hormone-binding globulin.
Perinatal
At birth, many of the sex hormone levels are similar in males and females due to fetal exposure from the mother. Progesterone (27) and estrogen levels (25) are high at first, but decline rapidly during the first few weeks to months of life (25,27). Similarly, testosterone concentrations nearly reach adult values in the perinatal period, thought to be secondary to maternal human chorionic gonadotropin (HCG) presence in the neonate bloodstream. When HCG clears, androgen concentrations decline rapidly (26). In both males and females SHBG is low at birth since androgens are high (26). GH is released in spurts and is difficult to test during the life span, therefore IGF-1 levels are typically measured and are low during this time period (28).
Prepuberty
During childhood, gamma-aminobutyric acid (GABA) inhibits the neurons in the hypothalamus that release GnRH causing low, relatively constant, levels of estrogen, progesterone, and testosterone (29;8, ch. 15). Possibly due to ovarian production, girls generally have slightly higher levels of estrogen and progesterone than boys during childhood (8, ch. 15;30). SHBG levels nearly double in girls and boys between ages 2 and 4, likely since androgen levels are low during this time (26). Both spontaneous and stimulated GH secretion increase during childhood, as demonstrated by linear bone growth and height (31).
Puberty
Puberty occurs between 8 to 14 years of age in girls and 9 to 14 years of age in boys (32). Average onset of puberty is age 11 and 13 in girls and boys, respectively. Puberty is determined by an increase in growth rate and physical sexual characteristics, described by the Tanner stages (33). During puberty, higher levels of excitatory amino acids such as glutamate increase the amplitude of GnRH pulses (29,34). In turn, FSH and LH pulses increase, leading to increased sex steroid output by the gonads (35). In both girls and boys, the initial rise in estrogen levels leads to a growth spurt (36;8, ch. 15). In girls, there is only a minor increase in testosterone while in boys testosterone increases rapidly and leads to the more obvious changes of virilization seen in males during puberty (26,35). In both sexes there is a continued decline of SHBG during sexual maturation. Both spontaneous and stimulated GH secretion increases during puberty, slightly earlier in females than males (31). Young adolescents secrete GH at almost 1.5 times the rate of healthy adults, and levels peak during teenage years (37,38). Serum relaxin levels during pubertal growth have been poorly studied. One study found that the majority of young men and women have undetectable levels of relaxin, but when relaxin is detectable, levels are comparable for both sexes (39).
Females Postpuberty
After puberty and before menopause, females experience cyclic changes in estrogen, progesterone, and relaxin within their menstrual cycle (Figure 1.7) while GH/IGF-1 downtrends slowly over the female life span (37).
There is a large peak in estrogen just before ovulation and a smaller, relative peak during the mid-LP (8, ch. 13). Concentrations of progesterone are lowest during menstruation and highest during the LP (8). These hormonal variations also have considerable intra- and inter-person variability (42). Relaxin levels have been reported to be low in the follicular phase (FP) (43) and peak during the luteal phase (44) due to production by the corpus luteum (45,18). This remains controversial as Pehrsson et al. reported that women have stable levels of relaxin throughout the menstrual cycle (46). Since Pehrsson et al. was the only study to collect and compare concentrations of all the sex hormones during the menstrual cycle, this study is used as the basis for Figure 1.7. However, it is important to note that the women in the Pehrsson study were in their 30s and many of them had been pregnant at least once, so the concentrations may not be translatable to the younger, athletic female. Mild rhythmic changes also occur with testosterone and SHBG during the menstrual cycle, with small increases seen during ovulation (26,41).
Males Postpuberty
The large increase in testosterone seen during puberty in males is followed by a sustained elevation until a decline after age 70 (26). Estrogen and progesterone concentrations remain relatively constant and low after puberty in males, similar to concentrations seen in women after menopause (26). GH/IGF-1 downtrends slowly over the male life span similar to females (37).
Females Postmenopause
The average age of menopause is estimated to be 50 to 52 years of age, but this varies with race, ethnicity, and lifestyle factors (47). In postmenopausal women, levels of estrogen and progesterone drop and become comparable to levels in men, remaining relatively constant and declining only slightly with age (8, ch. 13). Female testosterone concentrations continue to remain constant and significantly lower than male levels after puberty and for the remainder of the female life span (26). In women, SHBG slowly decreases between ages 30 and 70 (likely with regard to lower estrogen), but then rises again after age 70, the mechanism and significance of which is unclear (26).
FIGURE 1.7: Fluctuations of hormones during the menstrual cycle. Estrogen and progesterone levels are derived from NIH Clinical Lab value references. The values used are the mean values, derived from the ranges given via the cited reference. Testosterone values are from Ahrens et al. (40). SHBG and relaxin concentrations are derived from Pehrsson et al. (41). Please refer to the text for further explanation of this graph and possible variations in the literature.
COLLAGEN METABOLISM AND FUNCTION IN THE MUSCULOSKELETAL SYSTEM
Collagen is a basic building block of ligaments, tendons, cartilage, and bone. Collagen synthesis occurs in several steps and involves several cell types, including fibroblasts, chondrocytes, and osteoblasts (48). Polypeptide chains are translated, hydroxylated, and glycosylated in the rough endoplasmic reticulum. Three of these chains combine to form a procollagen molecule, which exits the cell through vesicular transport. Once the procollagen has left the cell, the propeptide extensions are cleaved, resulting in a tropocollagen molecule, the smallest distinct unit of collagen. Tropocollagen molecules aggregate to form microfibrils held together through relatively weak hydrophobic interactions. Microfibrils comprise five radially packed tropocollagens that are vertically staggered and form long chains with micrometer lengths and 3.5 to 4 nm diameters. Microfibrils form crosslinks with one another, forming fibrils with micrometer lengths and diameters between 30 and 150 nm (49). Fibrils combine to form fibers that have diameters that range from 1 to 10 micrometers. When collagen is organized into a multiscale structure it facilitates tissue function (48) (see Figure 1.8).
Collagen clearance and degradation occur by distinct mechanisms. Cleaved or intact collagen molecules can be internalized by urokinase plasminogen activator receptor-associated protein (uPARAP) receptors (50), while matrix metalloproteinases (MMPs) degrade fibrillar and nonfibrillar collagen, as well as the noncollagenous components of the extracellular matrix (ECM). More than 20 types of collagen have been identified. Collagen types I, II, and III are common types of fibrillar collagen (51). Type I collagen is the primary structural protein found in ligament, tendon, and bone. There are also nonfibrillar collagens found in basement membrane and ECM, including collagen IX, which is found in cartilage (48). Other collagen types of interest in sports medicine include type V, a fibrillar type that works with type I, and type XII, a fibril-associated collagen. Both are thought to provide structural support to ligaments (239). Variations in collagen genes may alter the structure of collagen types I, V, and XII, and this structural change might influence how ligaments respond to external load and risk of ACL injury (92,236,237).
FIGURE 1.8: Key steps of collagen synthesis: Formation of the collagen fibril within the fibroblast cell and levels of collagen fiber organization.
Collagen can function as compressive or tensile tissue, and these different functions require different structural arrangements. Bone is a compressive tissue that requires strength and some flexibility provided by a composite material of collagen and minerals (48). The collagen in articular cartilage is made of chondrocytes and is tightly packed to allow absorption of cyclic stresses that occur at the articular surface (48). The collagen in tendon and ligament is aligned parallel to the axis of tension to enable these structures to withstand tensile loading (52).
Collagen and the balance between collagen production and degradation are critical to musculoskeletal structure and function. Hormonal influences on collagen metabolism occur at a microscopic level, yet there is emerging evidence that these changes can have important macroscopic and functional implications.
HORMONAL EFFECTS ON TISSUES
Hormonal effects on tissues, including ligament, tendon, muscle, bone, and cartilage will now be reviewed in detail; it is summarized as a quick reference in Table 1.1.
Ligament
Receptors for estrogen (53), progesterone (53), testosterone (54), and relaxin (55–57) have been identified on human ligaments, and these hormones affect both synthesis and breakdown of collagen. An in vitro model employed by Yu et al. showed estrogen-dependent suppression of fibroblast proliferation, whereas progesterone seemed to attenuate estrogen’s effect on fibroblasts (58). Interestingly, estrogen’s effect on collagen may vary even within a specific ligament. Yoshida et al. showed that estrogen increased collagen III synthesis in the middle section of the ACL, but decreased collagen I synthesis in the proximal portion (59). DHT enhances the expression of androgen receptors and collagen synthesis through fibroblast proliferation in canine ACLs (60). Relaxin promotes collagen degradation by increasing MMPs, including collagenases, gelatinases, and stromelysins (61–65). Relaxin’s effect on MMPs is enhanced by estrogen (63), but attenuated by progesterone (63,66,67).
From the perspective of ligament function, high concentrations of estrogen have been shown to result in decreased stiffness of the human ACL (68) and decreased load to failure in rabbit ACLs (69). Prior work supports that relaxin also increases ligament and joint laxity (70–73) by reducing the density and organization of collagen bundles (74). The direct effect on human ligaments is unclear, but in guinea pigs, relaxin led to a 30% to 50% reduction in the strength the ACL (75). In addition, several studies have shown that markers of collagen metabolism (76), anterior knee laxity (77–79), and multiplanar knee laxity (80–82) fluctuate across the menstrual cycle.
However, there are several variables that limit our ability to understand how and which hormone(s) are driving these ligamentous changes. For example, there is a large intersubject variability in the timing and magnitude of sex hormonal fluctuations across the menstrual cycle (83). Furthermore, there may be a time lag of 3 to 5 days between the hormonal shifts and changes in ligamentous laxity (77). Finally, as described previously, sex hormones demonstrate a complex interaction, with estrogen enhancing the effect of relaxin and with progesterone modulating the effect of both estrogen and relaxin (54,58,84). Additionally, a lack of consistent methodology regarding how the hormone was administered, timing of measurements, and whether physiologic or supraphysiologic concentrations were administered further complicate our interpretation of the existing literature.
Tendon
Human tendons have been shown to have receptors for estrogen, relaxin, and testosterone (85–87). Estrogen increases tendon collagen synthesis by fibroblast-like cells called tenocytes (88,89) and has been shown to reduce stiffness of the hamstring tendon and the entire lower limb (90–93). However, other work has not demonstrated any change in patellar tendon stiffness across the menstrual cycle (94). Relaxin decreases the stiffness of patellar tendon in isolation (87), but this effect is attenuated by testosterone and enhanced by estrogen and progesterone (95). GH stimulates collagen accretion in dense fibrous tissue, with reduced collagen maturation (96). In addition, when GH was absent or in excess, there was decreased collagen fibril diameters (97). IGF-1 has anabolic effects on rat tendon fibroblast production of collagen, leading to tensile load-induced collagen formation and structural rearrangement (98–100). Furthermore, when IGF-1 is injected into injured rat tendons, there is acceleration of tendon repair (101). This information has some clinical applications that need further exploration. For example, when administering platelet rich plasma (PRP) as a treatment for tendinopathy or partial tears, platelets release IGF-1, which theoretically helps promote healing in ligaments and tendons, based on the literature (102). Another example that needs to be further explored is that males demonstrate an exercise-induced increase in aromatase and estrogen synthesis (12) and patellar tendon hypertrophy that is not seen in females (103). The mechanism of why patellar hypertrophy in exercise occurs only in males is unclear, but the study’s authors postulated a hormonally driven mechanism.
Muscle
Recall that steroidogenesis occurs in muscle in both sexes (12). Human skeletal muscle expresses receptors for estrogen and testosterone (104). DHT and testosterone increase muscle weight and mass independently from other hormones (105). GH/IGF-1 increases muscle mass by promoting sarcomere hypertrophy, increasing protein synthesis, and inhibiting protein degradation (3,106,107). IGF-1 also independently stimulates muscle stem cells (in response to mechanical loading on bone) to differentiate into myotubes (3) and induces muscle glycogen synthesis (20). Relaxin treatment in the muscles of mice increases MMP activation, increases satellite cell activation, increases angiogenesis, and decreases inflammation compared to untreated controls (108). Relaxin can also enhance muscle healing after injury in vivo. Supraphysiological doses of relaxin cause an increase in the number of regenerating myofibrils and decrease the area of fibrosis in damaged muscle (109). Direct effects of endogenous progesterone on muscle have not been observed in premenopausal women (92,94,110).
TABLE 1.1: Hormonal Effects on Tissues
The clinical applications of hormones on muscle in athletes are numerous. Concentrations of intramuscular steroid hormones are independent predictors of muscle strength in premenopausal and postmenopausal women (111). With regard to athletes building muscle mass, exercise increases endogenous GH levels to achieve sarcomere hypertrophy and increase protein synthesis in both sexes (112). Intramuscular steroidogenic enzymes, testosterone, DHT, and DHEA are also upregulated in skeletal muscle (113) with acute and chronic exercise stimulation leading to increased muscle mass (114). It has therefore been suggested that when in excess, a hyperandrogenic state could lead to performance enhancement (115,116). In fact, up to 11.1% of males report using performance-enhancing agents in their lifting routine (117). In women, Hagmar et al. reported overrepresentation of polycystic ovaries (higher free androgen index) in female Olympic athletes (37%) versus the general population (20%) (118). To recognize a hypoandgrogenic or hyperandrogenic state in women, the clinician must look beyond menstrual history alone. For instance, Rickenlund et al. (116) found that athletes with oligomenorrhea or amenorrhea did not always have associated hyperandrogenism. Those with hyperandrogenism (with or without menstrual abnormalities) did, however, have a higher anabolic body composition, higher total bone mineral density (BMD), higher maximal oxygen uptake, and higher performance values compared to those without hyperandrogenism (116). On the opposite end of the spectrum, reductions in testosterone in men can trigger a decline in muscle mass (119).
Given the importance of androgen effects on muscle and possibly performance, serial serum androgens concentrations, including SHBG, are being explored as a way to evaluate performance and establish normal values for elite athletes (120). For example, these profiles have shown lower testosterone concentrations and higher SHBG levels in athletes taking exogenous hormones such as oral contraceptives (118,120,121). The significance of this is very important for clinicians treating athletes, as almost 47% of Olympic athletes in 2009 were hormonal contraceptive users and, based on the literature, may be at an athletic disadvantage (118). Oral contraceptives are further discussed in this chapter in the section on effects of hormonal contraceptives on the musculoskeletal system, injuries, and performance. In treating hypogonadal states, hormone replacement therapy (HRT) has been demonstrated to be useful. Selective ligands and selective androgen receptor modulators (SARMs) have been shown to maintain the positive effects on muscle repair (122). Postmenopausal women on HRT also demonstrated greater muscle strength and mass than their untreated twins (122).
Another important clinical application of hormonal influence on muscle is demonstrated by studying muscle function during the menstrual cycle. There are discrepancies in the literature on whether changes in muscle strength and function occur in the quadriceps and hamstrings with regard to the menstrual cycle, likely again with regard to how hormones were measured and how each phase of the menstrual cycle was determined (123). Sarwar et al. found that muscle strength varies throughout the menstrual cycle, with a peak in quadriceps isometric torque at mid-cycle when estrogen levels are highest. These authors also found increased fatigability and longer relaxation time at mid-cycle (124). Bell et al. also showed that estrogen is negatively correlated with the rate of force production in women during the early FP (92). Bambaeichi et al. found that peak knee extensor torque occurred on a day near ovulation while peak knee-flexor torques occurred on a day in the ovulatory phase (125). However, Kubo et al. showed stable levels of muscle strength, peak torque, and relaxation time despite fluctuations in progesterone and estrogen levels across the menstrual cycle (94). Gur also found no change in concentric or eccentric muscle torques of the quadriceps and hamstrings throughout the menstrual cycle (126).
Clinically, the effects of GH on muscle are better understood by examining GH-deficient patients. GH-deficient patients demonstrate reduced muscle mass and reduced BMD, but with exogenous GH supplementation, skeletal muscle mass increases in the first 12 months of therapy (127). Athletes are aware of the effects seen with growth hormone administration in deficient children and adults. Therefore, healthy power athletes often seek GH supplementation to enhance performance. However, it has not been definitively shown that healthy athletes will benefit from GH treatment like deficient patients. For example, in experienced weightlifters, amino acid incorporation into skeletal muscle protein was not increased and the rate of whole body protein breakdown was not decreased with GH administration. In another study of exogenous GH in power athletes, GH did not increase maximal strength in the biceps or quadriceps (128). Contradictory to this finding, when exogenous GH was given to a portion of healthy men over 50 years of age, there was a significant increase in leg press in the GH group (129). Another study showed that with supraphysiologic doses of GH to athletes, there was reduced oxidative protein loss during exercise (130).
Bone
Bone development and maintenance is a multihormonal phenomenon. Bone growth increases during childhood through puberty, with puberty being the final stage in bone growth (131). During puberty there is a rapid and significant growth rate increase called the acceleration phase. During this phase, there is an increase in standing height velocity of greater than 6 centimeters/year in girls and more than 7 centimeters/year in boys; this acceleration phase typically last about 2 years and is followed by a deceleration phase (33). The hormone estrogen has a critical role in this bone development and also in bone maintenance. The initial rise of estrogen in early puberty is needed for linear growth of bone during puberty, and the high levels of estrogen in late puberty are required for growth plate closure in both males and females (8, ch. 6;36). Free estrogen is a positive predictor of cortical volumetric bone marrow density (132). For bone maintenance, estrogen triggers apoptosis of osteoclasts, leading to reduced degradation of the bone (133). Furthermore, when estrogen decreases and osteoclast activity increases, the net result is degradation and resorption of the bone (134). Meanwhile, androgens increase calcium absorption and retention and help determine bone width and cortical bone growth (34,132,135). This is further supported by bone length being unaltered in the absence of androgen receptors (135). Progesterone helps stimulate bone growth and maintain established bone mass in concert with other hormones (238). GH/IGF-1 is largely responsible for enhancing cortical bone mass via estrogen and mechanical bone strength (136). During puberty, boys acquire more bone mass at the periosteum than girls (137), a process which relies on GH/IGF-1 regulation of estrogen (138). GH also contributes to bone length, as deficiency leads to short bone length (139). The influence of relaxin on human bone is unclear, but in vitro and in vivo animal experiments have provided clues about its possible effects. In vitro experiments have shown that relaxin stimulates osteoclastogenesis from hematopoietic precursors (140, 141). Relaxin may also help stimulate osteoblast differentiation and bone formation through bone morphogenic protein (BMP)-2 in vitro (142).
Clinically, the amount of estrogen in males and females and the timing of puberty are very important in both sexes in determining lifelong bone health and fracture risk. For example, excessively high levels of estrogen during puberty can lead to premature hardening of the epiphyseal cartilage and short stature (8, ch. 6;36). Furthermore, in men with estrogen resistance, there is continued linear growth of the bone and the density and strength of the bone are compromised (36). Women who achieve menarche at age 15 or older are at an increased risk of fracture due to reduced bone strength after menopause (143). Low serum estrogen can predict incident fractures, and this risk increases with low testosterone and high SHBG (144). Female athletes have been shown to have a higher incidence of stress fractures compared to male athletes (145). This increased fracture risk associated with late menarche, or primary amenorrhea, may result from disturbances in estrogen levels seen with energy deficiency associated with the female athlete triad (FAT), which may lead to a decrease in bone cortical thickness, an increase in trabecular area, and a deterioration in bone microarchitecture (143,146). Chapter 12 on bone health has further details regarding the FAT and stress fractures.
Over the past decade, there has also been a statistically significant increase in the incidence of radial and carpal fractures seen in girls and boys age 10 to 14 as compared to children age 5 to 9 and adolescents age 15 to 19 (147). The study extrapolates that this increase is due to increased participation in sports. While this may certainly be the case, one must also consider that the varying factor for that age group compared to the others may be the hormonal changes occurring during peak growth phases, like that seen in puberty with high bone turnover and growth (147). Ongoing research is needed to determine the clinical utility of serum sex steroids for fracture prediction (144).
Cartilage
Sex hormone receptors have been found on animal and human chondrocytes, and many studies have focused on their role in cartilage protection (148). Interestingly, the hormonal implications on cartilage appear to be sex specific (149). Claassen and others demonstrated that estrogen protects chondrocytes by providing an antioxidative effect (150,151). Kenny et al. demonstrated that only female rat chondrocytes respond to estradiol supplementation, despite both males and females having estrogen receptors (152). Similar to estrogen in females, testosterone appears to only affect male chondrocytes (153). Higher serum testosterone is associated with achieving a greater amount of knee cartilage over short periods of time. Longitudinally, however, higher free testosterone correlates to an increased rate of cartilage loss (148). Estrogen also suppresses matrix metalloproteinases (MMPs) in articular chondrocytes from female patients but not in male patients (150). Relaxin leads to degradation of cartilage by inducing MMPs (62,64). The degenerative effect of relaxin on MMPs may contribute to the progression of osteoarthritis (OA). One study found that the intensity of immunostaining for RFXP1 correlated to the severity of OA (154). The effects of relaxin on MMPs can be enhanced by estrogen and attenuated by progesterone (62,63). GH also has direct effects on chondrocytes, stimulating division and multiplication of chondrocytes in cartilage (155). In a study with human articular cartilage pretreated with a cytokine (that stimulates cartilage degradation and chondrocyte apoptosis), there was rescued matrix biosynthesis when treated with IGF-1 (156).
Clinical studies examining the relationship between endogenous hormones and OA in humans suggest there may be association between endogenous estrogen and cartilage protection, but the evidence is inconsistent (157). A large cohort study focused on the relationship between endogenous sex hormones, laboratory levels, and radiographic OA. The study examined radiographs and determined that subjects with low estradiol levels had increased risk of OA incidence (158). Those with low 2-hydroxyestrone (a breakdown product of estradiol catabolism with weak estrogenic activity) were at greater risk for both incidence and prevalence of knee OA. The study proposed that higher levels of 2-hydroxyestrone may delay the development of knee OA by inhibiting leukotrine synthesis (158). Another study that measured serum estrone, estradiol, testosterone, and androstenedione determined concentrations of hormones do not affect OA severity (159).
There do not appear to be any studies examining the effect of sex hormones and other cartilage lesions such as osteochondritis dessicans or chondromalacia patellae.
Nervous System
The role of hormones on the central and peripheral nervous system is less understood when compared to joints, ligaments, muscle, and tendon. GH and IGF-1 are both critical for neural development. They regulate the size, morphology, and function of cells of the central nervous system (CNS). GH treatment affects neurogenesis, myelin synthesis, and dendritic branching and can activate a subpopulation of neural stem cells (160). IGF-1 can improve regenerative capacity of motor neurons in both the central and peripheral nervous systems (156). Nuclear and membrane receptors for progesterone, estrogen, and testosterone also exist in the nervous system (161–163). Estrogen has an excitatory effect on neural substrates in the brain and spinal cord (164,165). Meanwhile, progesterone likely plays an inhibitory role in the CNS (166). In addition, nuclear progesterone receptor expression increases with estrogen exposure, indicating potential interactions between these hormones in the brain (161). Although the mechanism is unclear, progesterone may influence the perception of pain. Locally synthesized progesterone can help reduce the perception of pain in the CNS by upregulating endorphins and opioid receptors (167). In the nervous system, progesterone also seems to have an anti-anxiety effect through the gamma-aminobutyric acid (GABA) receptor, specifically GABA-A (6). Testosterone has been shown to increase cell excitability, attenuate atrophic changes, and improve the regenerative capacity of motor neurons (163). In the brain, relaxin acts as a novel neurotransmitter with numerous functions, affecting spatial memory, stress response, food intake, the sleep-wake cycle, and arousal (168). Relaxin may also be involved in energy balance in the CNS and reproductive signaling (169).
More clinically focused studies have examined different measures of motor control across the menstrual cycle, but differences in methodology and lack of daily testing have resulted in several studies demonstrating changes (170–173) while others have not (174–176). Two recent studies that focused on more basic measures of neuromuscular control demonstrated changes across the menstrual cycle: Casey et al. detected a decrement in the muscle stretch reflex measured at the rectus femoris during the time around ovulation (177), while Tenan et al. demonstrated changes in vastus medialis firing and control during the menstrual cycle (178). Finally, Bonifazi et al. observed increased corticospinal excitability in the setting of supraphysiologic levels of estrogen in males (179). The findings in these studies warrant future investigation regarding the underlying mechanisms of change as well as the origin along the neuromechanical axis.
EFFECTS OF HORMONAL CONTRACEPTIVES ON THE MUSCULOSKELETAL SYSTEM, INJURIES, AND PERFORMANCE
Exposure to exogenous estrogen and progesterone in the form of hormonal contraceptives is common in active females. Approximately 100 million women worldwide and up to 70% of collegiate athletes in the United States report using hormonal contraceptives (180,181). Given such a high prevalence of hormonal contraceptive use in athletes, it is imperative that the musculoskeletal and physiological effects are well understood so that clinicians and athletes can consider the impact of these medications on injury and performance. This section reviews the current state of knowledge and will pose critical questions for future investigation.
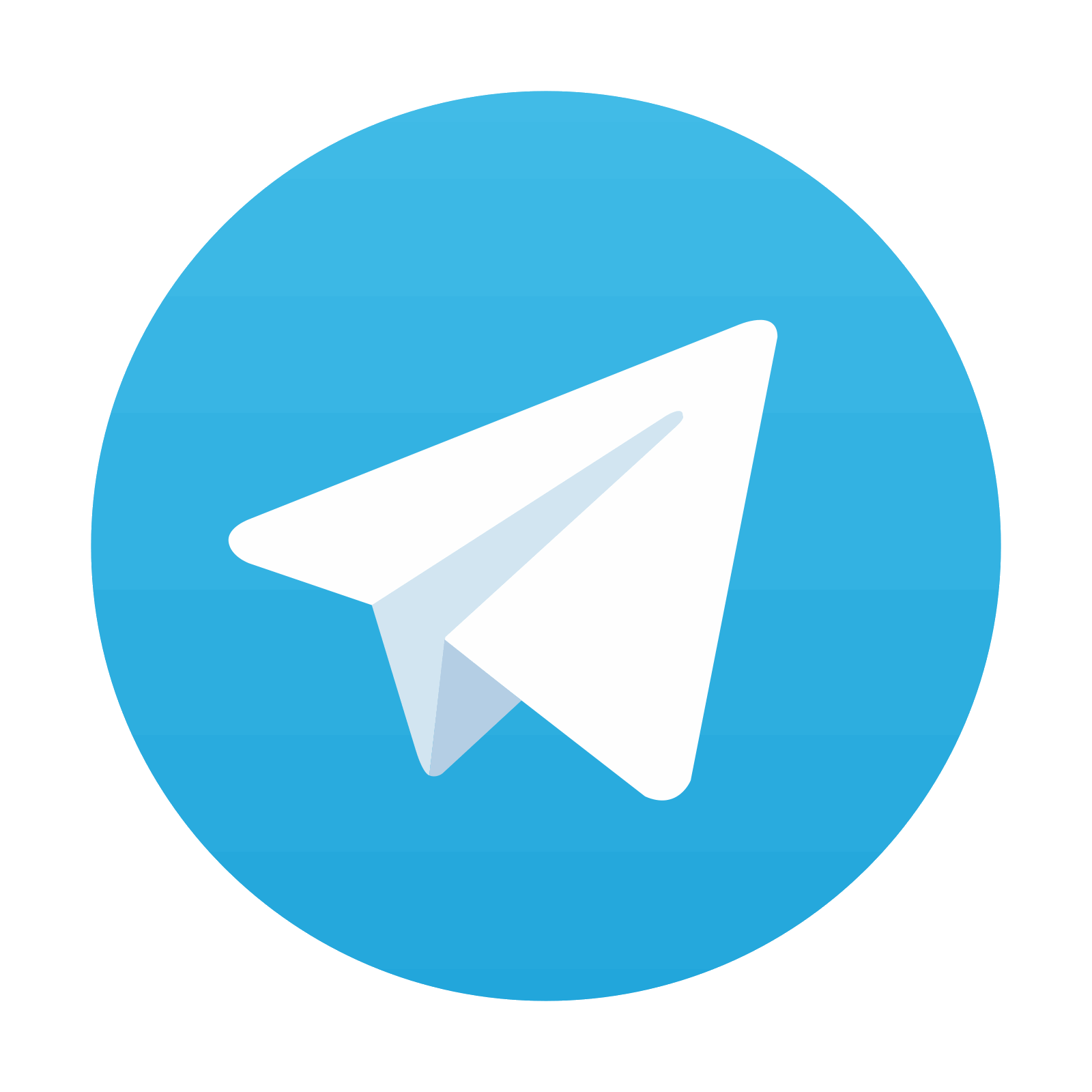
Stay updated, free articles. Join our Telegram channel
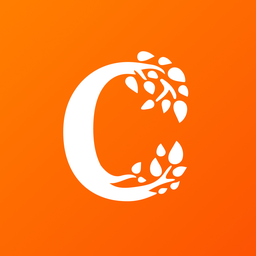
Full access? Get Clinical Tree
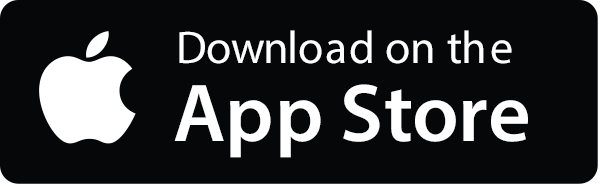
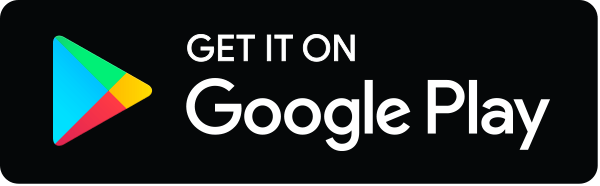