Summary
Scoliosis was classically seen as only a coronal plane deformity, but we now know this deformity is much more complex and exists in multiple planes. Continual development of deformity correction instrumentation has occurred since the early 1960s, with the goal of preventing further scoliosis and, if possible, straightening the spine. Correction is traditionally achieved through arthrodesis by the mechanics of applying force to correct or reduce the correction and creating an environment for fusion to occur by immobilization. Innovation has led pedicle screw–based correction techniques to become the basis for correction constructs. These constructs vary widely in screw pattern and technique, which leads to different mechanical forces that need to be considered. Loads applied to instrumentation are highly variable, and corrective constructs exert forces that can lead to failure by way of screw pullout and rodor screw breakage, whereas failed fusions eventually lead to loosening or breaks of instrumentation. An undesirable consequence of spinal instrumentation is proximal junctional kyphosis and the more severe form, proximal junctional failure. The understanding of deformity correction mechanics has progressed along with the instrumentation itself, and the use of computer-based biomechanical modeling offers the ability to explore surgical options and understand instrumentation biomechanics.
Key words
scoliosis – biomechanics – deformity instrumentation – failure – correction – force – pedicle screw7 Biomechanics and Correction of Scoliosis
7.1 Introduction: Three-Dimensional Morphology of Scoliosis and Spine Biomechanics
Scoliosis was classically defined as a lateral curvature of the spine, the severity of which is measured clinically by the Cobb angle, which is the angle between the lines drawn on the end plates of the most tilted vertebrae in the radiographic curve. However, the deformation of scoliosis is much more complex with a three-dimensional (3D) anomaly of the axial skeleton, with rotation of the vertebrae and rib cage. 1 The rotation of vertebral bodies is usually on the convex side, generally accompanied by a rotation of the back surface in the same direction, producing a “rib prominence” (gibbosity). Transverse rotation is most important at the apex of the curves (the levels of the most laterally deviated vertebrae), but the associated back rotation could be a few vertebrae lower than the apex in the thoracic and thoracolumbar curves. Asymmetries of the vertebrae (wedging, disproportion of the pedicles, deformation of the posterior processes) also develop as a result of altered stresses on the bones, as well as disks, probably as the primary site of deformation and then as a compensation mechanism. The deformation of the transverse plane is also accompanied by a modification of the pelvis and of the sagittal profile, often with a paradoxical reduction of thoracic kyphosis and lumbar lordosis (LL).
The geometry of the spine can also be considered a curved spline in space with vertebrae positioned along this vertebral body curve. The vertebral body curve in turn defines planes of maximum curvature. The former Scoliosis Research Society (SRS) 3D Scoliosis Committee defined the rotation of the planes of maximum deformity as the angle between the sagittal plane and the planes passing through the apex and end vertebrae of each scoliotic curve. 1 , 2 In a straight, healthy spine, the planes of maximal curvature are in the sagittal plane, but in a scoliosis the planes of maximum deformity are rotated due to the lateral deformity and the reduction of the sagittal curves.
7.2 Deformity Correction Mechanics
In the case of severe spinal deformities (Cobb angle > approximately 50 degrees), surgical spinal fusion with implants is often performed. The goals of surgical treatment are to prevent further worsening in the scoliosis and, if possible, to straighten the spine. This is normally achieved through arthrodesis (bony fusion) of a region of the spine that spans one or more scoliosis curves. Implants used in the surgical treatment of spinal deformities have two complementary mechanical roles. The first is to apply forces to correct or reduce the spinal deformity intraoperatively and to maintain correction subsequently. The second is to create the correct mechanical environment for spinal fusion to occur by immobilizing the spine until bony fusion has occurred. Instrumented spinal fusion remains the primary treatment approach for the management of severe scoliosis.
Originally, scoliosis was initially viewed as a coronal plane deformity. Consequently, the initial efforts were directed at coronal plane correction. The initial work was directed at using a cast to help correct the deformity and then a fusion was done through a window cut into the cast. 3 , 4 The challenges with this technique were the skill required to apply the cast, the use of prolonged recumbency, limited ability to correct the deformity, and high pseudarthrosis rates.
The first major advance of spinal instrumentation was the Harrington rod. Initially, Harrington used this in patients with polio and did not do a concomitant fusion. This led to a very high rate of dislodgement. Moe advocated for simultaneous fusion and instrumentation. This led to improved correction and fusion rates but still required postoperative casting to avoid hook dislodgement. 5 The concept of Harrington instrumentation was twofold. First, distraction was applied in the concavity, which, for curves greater than 50 degrees, was the most efficient form of correction of the scoliosis. Second, as an adjunct, convex compression rods aided the correction (Fig. 7‑1). For very large curves, the concept of stacked rods in the concavity enhanced the correction as well. There were some significant limitations of Harrington rods. The first was that distraction-induced relative kyphosis and lordosis reduction and the scoliosis correction came at the cost of flattening the sagittal curves. The Moe modification involved a square-ended rod and hooks that allowed sagittal contour to be applied and better maintained. This was widely accepted. There was still the problem of hook dislodgement, and Bobechko developed a dual hook system to minimize this problem. 6 This was not as widely adopted.

With the initial introduction of the distraction technique, there was a significant increase in the rate of neurologic deficits, probably from overdistraction. This led to the initial development of the SRS Morbidity and Mortality efforts to try to identify and improve upon this problem. 7 Subsequently, the wake-up test was developed by Stagnara as a way to assess the neurologic status intraoperatively. 8 Subsequently, neuromonitoring techniques were developed by Nash et al. 9
The next significant advance was the use of sublaminar wires popularized by Luque 10 (Fig. 7‑2). In his practice in Mexico, keeping kids in casts in the summer was problematic. Sublaminar wires obviated the need for postoperative casting. Originally, this technique used L-shaped rods that were cantilevered into place, and lateral translation through tightening of the wires provided the correction. This technique was quite efficient at correcting deformity, and the implants were inexpensive as well. Again, with initial adoption, there was an increase in neurologic deficits, which improved with better understanding of the technical aspects of passing the sublaminar wires. An additional modification was the development of spinous process wiring using buttons to avoid the need for spinal canal entry and lessen the neurologic risk. 11

The use of sublaminar wires was then combined with Harrington distraction instrumentation, which provided additional incremental improvement and efficiency in the correction of the deformity.
Cotrel and Dubousset developed a device for transverse traction (DTT) that pulled the convex compression rod to the concave distraction rod, improving the lateral translation correction. The Cotrel–Dubousset (CD) instrumentation technique was the standard of care for approximately 20 years. Cotrel and Dubousset also popularized the use of hook claws with their new instrumentation system 12 , 13 (Fig. 7‑3). Previously, hooks could only apply unidirectional force (either distraction or compression). With hook claws, distraction and compression could be variably applied to the hook claw. This changed the instrumentation pattern and the deformity correction process. They introduced the concept of rod derotation maneuver especially, which was originally thought to derotate the spine and translate it to correct the deformity. Subsequently, it was found that the rod derotation maneuver really only applied a lateral translation force. 14 The CD system again did not require postoperative immobilization and appeared to give enhanced correction. The thinking process involved in fusion level selection was more complicated, and the phenomenon of decompensation showed up when the technique was not perfectly applied. This gave rise to significant efforts to better understand the true 3D nature of the deformity and critically look at ratios of curves for rotation and translation. 15

In this time frame, pedicle screws began to become more widely used. Steffee plates were popularized in the United States and were widely accepted. 16 Closed head screws were available with CD instrumentation but were technically challenging to use. Barr et al 17 showed improved lumbar curve correction with the use of pedicle screws. Asher et al 18 developed the ISOLA system, which allowed the use of hooks, wires, and screws within the same system. This allowed more specific force application by level. Other systems such as the Texas Scottish Rite Hospital (TSRH) system were near contemporaries and allowed similar force application. 19 An initial limitation of all of these systems was that the screws had fixed connections or fixed heads making their use more technically challenging. 12 , 20 Subsequently, Puno and Byrd 21 developed a polyaxial screw system that made it easier to make the connections. This was initially a nonlocking polyaxial head. Subsequent iterations led to poly- or multiaxial screws that when the capturing device was tightened became locked. For the thoracic spine, the correction was primarily still done with hooks or wires. In 1995, Suk et al 22 published the first clinical series using thoracic pedicle screws. Although there was initial skepticism about the safety of the technique, subsequently it has become the dominant anchor pattern in use today. Initially, there were learning curves for screw placement. Additional technologies were developed to enhance the accuracy of screw placement (electrical stimulation of the screws, computed tomography [CT] based navigation systems, etc.). 23 , 24 The use of pedicle screws allows true 3D force application (compression, distraction, derotation, translation) to each segment. 25
7.3 Growth Modulation Instrumentation Mechanics
In adolescent idiopathic scoliosis (AIS), the progression of scoliosis is associated with the rate of skeletal growth. As scoliotic deformation progresses, vertebrae are increasingly unevenly loaded, resulting in an asymmetric distribution of stresses and then growth rate in vertebral growth plates. This acceleration of deformation associated with mechanically modulated growth has been described as a “vicious circle” 26 that begins after a certain threshold of deformation of the spine has been reached. This concept of a biomechanical mechanism of deformation progression has been incorporated into the rationale for orthopaedic braces and surgical fusionless treatments. Such treatments aim to modify the asymmetrical loads to stabilize or reverse the deformity progression using growth modulation.
One of the recently introduced techniques is based on anterior vertebral body growth modulation. It is a fusionless procedure that consists of instrumenting the convex side of the scoliotic curve with screws connected by a flexible link that applies a compressive force between each pair of vertebrae, which thereafter modulates the vertebral growth while permitting spinal movement. Observed correction after 2 years is reported to be between 45 and 75%. 27 , 28 , 78 A planning tool based on a patient-specific finite element model (FEM) of pediatric scoliosis integrating growth allows us to predict immediate to 2-year postoperative correction within 3 degrees of Cobb angle. 28
7.4 Multisegmental Correction Mechanics and Failure Modes
Pedicle screws have become the state-of-the-art fixation constructs for spinal fusion surgery. Anchored into the strongest part of the vertebral bony structure, they provide higher corrective forces and better 3D correction compared with hooks and hybrid constructs. 22 , 29 , 30 Pedicle screw–based correction techniques, such as segmental and direct vertebral derotation, have been developed and refined to optimize deformity correction in the three anatomical planes. 31 , 32 With more and more surgeons using predominantly pedicle screw constructs, wide variation in clinical practice was noted regarding the screw pattern (i.e., screw distribution and number) as well as the surgical techniques. 33 , 34 , 35 , 36 , 37 The reported mean screw density varies from 1.04 to 2 screws per level with percent curve corrections from 64 to 70%. 35 Some surgeons routinely use bilateral screws at every vertebral level included in the fusion (density of 2), whereas other surgeons use up to 46% fewer screws. 36 , 37 Improved curve correction has been reported with high versus low screw density in some studies, 36 , 37 but in other studies, the difference between the high and low screw density groups was not significant. 38 , 39 , 40 Constructs of high screw density have been associated with increased operative time, blood loss, radiation exposure, instrumentation costs, and risk of screw-related complications. 41 , 42 , 43 , 44 , 45 Since using constructs of low screw density may have benefits for optimal use of health care resources, 35 , 36 , 46 alternative screw patterns have been proposed. 47 Clinical studies on patient-specific optimal screw patterns are limited by the fact that different screw patterns cannot be tested on a given patient. Scientific data on the relative biomechanical performances and advantages of high versus low screw density constructs are still insufficient; screw density is mainly based on each surgeon’s knowledge, experience, and preferences. 34 , 37 , 47
The rationale of using constructs of high screw density is that more screws may help improve 3D correction and reduce the risk of fixation failure by spreading corrective forces over more anchor points. Computational studies showed that the 3D forces acting on the spine could significantly vary with construct designs and correction techniques. 25 Biomechanical analyses also demonstrated that only a portion of some of the components of these 3D forces contributed to the deformity correction, whereas the rest were due to the incompliance between the rod contour and the screw alignment. We distinguish, therefore, “true corrective forces” and “extra forces.” The former are the minimum forces required to achieve the desired corrections independent of the requirement that rod should be properly seated at all pedicle screws; the latter are supplementary forces to align all screws onto the rod, overcoming the imperfection in the screws’ alignment without improving the curve correction (Fig. 7‑4). Instrumentation simulations using computer biomechanical models of 10 AIS cases revealed that the average true corrective forces were 50 ± 30 N, but the actual bone–screw forces were 229 ± 140 N when using monoaxial (fixed-angle) screws and 141 ± 99 N when using polyaxial (multiaxial) screws. 25 It was noted that screw density had opposing effects; higher screw density may allow better load sharing among anchor points but may induce overconstraint and extra forces at the bone–screw interface.

Typical alternative screw patterns in scoliosis instrumentation showed similarity in terms of deformity corrections in the three anatomical planes but not for forces at the bone–screw interface. 48 For instance, a reference screw pattern (two multiaxial screws with dorsal height adjustability at every level fused) and four alternative patterns were compared. 49 In the alternative patterns, screws were dropped on the convex or concave side at alternate or periapical levels to have 21 to 25% fewer screws than the reference pattern. The simulated correction technique was simultaneous concave and convex segmental translation. The final simulated Cobb angle differences with the alternative patterns varied between 1 and 5 degrees for most simulations compared to the reference pattern. Thoracic kyphosis and apical vertebral rotation were within 2 degrees of the reference pattern. Bone–screw forces for the alternative patterns were higher than the reference pattern (p < 0.05). There was no statistical bone–screw force difference between convex and concave alternate dropouts and between convex and concave periapical dropouts (p > 0.05), and alternate dropout screw forces were higher than periapical dropouts (p < 0.05). It was concluded that using a simultaneous segmental translation technique, deformity correction can be achieved with 23% fewer screws than in a maximal density screw pattern but with up to 25% higher bone–screw forces. Screw dropouts could be either on the convex side or on the concave side at alternate levels or at periapical levels. Periapical screw dropouts may more likely result in lower bone–screw force increase than alternate level screw dropouts.
In another study, 140 posterior instrumentations were computationally simulated to compare the reference and alternative uniaxial screw patterns in AIS instrumentation with segmental translation and apical vertebral derotation techniques. 48 Compared to the reference pattern, alternative screw patterns allowed similar (<4 degrees) corrections in the coronal and sagittal planes, but higher screw density in the apical region (apex ± 1 level) had better percent apical vertebral rotation correction (r = 0.887, p < 0.05). Bone–screw forces with the reference pattern were 243 ± 54 N and those with the alternative patterns were 11 to 48% lower; high screw density tended to overconstrain the instrumented spine and resulted in higher forces at the bone–screw interface.
In addition to screw density, rod stiffness and contouring are other important contributing factors to the deformity correction and bone–screw forces. Differential rod contouring technique is used to achieve vertebral rotation correction. A computer biomechanical study showed that increasing the concave rod contouring angle and diameter with respect to the convex rod improved the transverse plane correction but with significant increase of screw pullout forces and thoracic kyphosis. 50 For instance, increasing the concave–convex differential rod contouring angle from 25 to 60 degrees, the apical vertebral rotation correction increased on average from 35 to 68%, the coronal plane correction changed from 76 to 72%, the thoracic kyphosis creation from 27 to 144%, and screw pullout forces from 94 to 252 N. Increasing the concave rod diameter from 5.5 to 6 mm resulted in increased transverse and coronal plane corrections, higher thoracic kyphosis, and screw pullout forces. The 3D corrective force patterns at the apical levels in differential rod contouring are illustrated in Fig. 7‑5.

Spinal instrumentation constructs are subject to intraoperative and postoperative loads under which rod breakage and pedicle screw fixation failure may occur. 51 , 52 To reduce the risk of rod breakage, there is increasing discussion on using auxiliary rods to share the loads and reduce stresses in the primary rods. Auxiliary rods could be added over multiple vertebral levels where higher bending loads are anticipated in the primary rods, such as where a pedicle subtraction osteotomy is performed. Auxiliary rods could be connected to the instrumentation using various types of connectors as well as with special screws allowing to connect two rods. In-house computer simulations using a biomechanical model were performed to compare the traditional bilateral rod construct with auxiliary rods. The results revealed that, overall, the reduction in primary rod bending moments ranged from 17 to 48% for standing loads as well as under simulated 8-Nm functional moments in flexion and extension.
7.5 Bone Implant Interface Failure Mode (Pullout and Nonaxial Loads)
Pedicle screws for spinal deformity instrumentation are subjected to variable loads in different directions due to various surgical maneuvers. 20 , 25 During direct vertebral derotation techniques, lateral or medial forces are applied to correct the transverse plane deformity, whereas when distraction and compression maneuvers are performed, cranial or caudal forces are applied. 12 To reach correction goals without fixation failure, the screws require an adequate purchase, 53 and safe force levels have to be exerted during the correction maneuvers.
Screw fixation is affected by the pedicle shape and size as well as the bone characteristics of the patient, such as the bone density and cortical bone thickness. The pedicle width and the cortical bone thickness 54 can be significantly diminished on the concavity of scoliotic curves, and pedicle screws must then be used with caution. 55 , 56 Chou et al also identified a significant correlation between cortical bone area ratio (cortical bone area over pedicle area in the pedicle isthmus) and the thoracic pedicle screw pullout strength in axial loading conditions. 57
Screw type and size, entry point preparation, tapping diameter, bone engagement (unicortical or bicortical) or screw head hubbing, and screw trajectory also affect the screw purchase. Paik et al 58 and Bianco et al 59 showed that the iatrogenic fractures caused by the head hubbing method weakened the cortical bone around the entry point and caused around 40% decrease of axial pullout strength. The use of larger diameter screws is known to significantly increase the pullout fixation strength. 60 , 61 Bicortical screws (inserted with anterior cortical wall violation) were considered an alternative approach with potential to generate more rotational torque and less bone fracture as it has been shown to provide 40% higher force to failure than unicortical screw placement under lateral loads. 59 , 62
7.6 Long-Term Failure (Cyclic Loading)
Spinal fusion is and always has been a race between biology and biomechanics. If fusion fails, eventually all spinal instrumentation either loosens or breaks. The spine cycles between 1 and 10 million times a year. The loads applied to the instrumentation are highly variable depending on the location (cervical, thoracic, lumbar, or sacrum/pelvis), patient factors of weight, activity and bone density, and the construct design (anchor density, anchor rigidity, use or absence of anterior column support).
Typical failure modes are somewhat predictable. For longitudinal members, rods will fail at the maximum stress concentration point. If the rod has a size change, where it reaches its smallest diameter is the likely point. This was seen in the ratcheted portion of Harrington rods and is also seen in transitional diameter rods. Otherwise, the maximum strain is often at the site of a pseudarthrosis. Commonly, these are at the thoracolumbar junction and the lumbosacral junction. Rod diameter and material also play a role.
For hooks, the usual failure mode is dislodgement. For sublaminar wires, the failure mode is pull-through or cutout of the lamina. Presumably, sublaminar bands would follow a similar pattern, although their wider, more flexible nature may change that. Screws either loosen or break. Loosening is seen as halo formation and is probably more likely in capacious pedicles (such as L5) where the fit and fill will be less. Breakage is dependent on the screw design. Tapered shank screws typically break where the shaft reaches its smallest inner diameter. In polyaxial screw designs, the failure is most typically at the head–screw junction.
Cyclic loading testing is challenging. When done in anatomic tissue, there is significant degradation after 8 hours. Also, there is no biological response, either positive or negative. The alternative ASTM International (formerly known as the American Society for Testing and Materials) standards utilize polyethylene cylinders and presume a perfect anchor–bone interface. This does, however, allow anchor design and construct variation to be tested. These models for long construct testing are nontrivial, as applying sagittal contour to the models makes them intrinsically unstable. However, some good generalizations can be confirmed with these models. Increasing the number of anchors increases the overall construct stiffness, regardless of the rod diameter. Sagittal contour (increasing kyphosis) has a significantly negative effect on construct stiffness. Anterior column load sharing has a markedly positive impact on construct stiffness. Optimal construct stiffness is unknown. A construct that is too stiff results in decreased load sharing and may limit fusion mass development and maturation. A construct that is too flexible results in pseudarthrosis formation or early fatigue failure. With the development of sophisticated finite element modeling and finite element analysis (FEM/FEA), greater insight can be gained from the FEM/FEA efforts. 59 , 60
7.7 Complex Deformity Correction Problems (Proximal Junctional Kyphosis and Proximal Junctional Failure)
One of the surgical objectives for the treatment of sagittal deformity is to restore normal sagittal balance. A wide variation exists in sagittal spinal alignments, which are considered normal; knowledge on how mechanical stresses are distributed in spines of normal alignment is essential to determine patient-specific surgical strategies. One of the undesirable consequences of spinal instrumentation is proximal junctional kyphosis (PJK), a pathological kyphotic deformity of the spinal segment proximally adjacent to spinal instrumentation. It generally has a limited clinical impact and is often considered the body’s natural response to the instrumented spinal fusion. Based on the severity of PJK, a subset of patients has been identified with a more severe form of PJK, which was referred to as proximal junctional failure (PJF). 63 , 64 The pathologic changes to PJF may occur early after the initial surgery and can be in the forms of compromised structural integrity, neurologic deficit, 62 topping-off syndrome (junctional compression fracture, subluxation, retrolisthesis, focal kyphosis, and disk height loss), 65 PJ acute collapse, 66 and fractures at the top of long segmental instrumentation constructs, 67 and would need to be addressed through revision surgery. Advanced age, osteopenia, preoperative comorbidities, sagittal balance, fusion to the sacrum, level of upper instrumented vertebra (UIV), PJ dissection, and use of pedicle screw at the UIV have been associated with the risks of PJF. 63 , 66 , 67 , 68 , 69 , 70 , 71 , 72 , 73 , 74 A retrospective review of a large adult spinal deformity database revealed that the strongest PJF predictors were age, lower instrumented vertebra (LIV), UIV, preoperative sagittal vertical axis (SVA), implant type at the UIV, preoperative pelvic tilt (PT), and preoperative difference between pelvic incidence (PI) and LL. 75 Based on these baseline demographic, radiographic, and surgical factors, a computer expert system–like model was built that can predict PJF and clinically significant PJK with 86% accuracy based on 510 adult spinal deformity patients with 2-year follow-up. 75 However, biomechanical studies on individual identified risk factors are very limited. The pathomechanisms of PJK were studied using numerical modeling and design of experiment techniques, 76 , 77 which provided useful biomechanical facts by assessing the resultant PJ force, moment, and kyphotic angle as functions of UIV, proximal implant type, osteotomy procedure, transition rod diameter, sagittal balance, and sagittal rod curvature. The PJ angle, proximal moment, and force were reduced by 18, 25, and 16%, respectively, when one more proximal vertebra was included in the fusion. When posteriorly shifting the sagittal balance by 20 mm, the PJ angle, proximal moment, and force increased by 16, 22, and 37%, respectively. Bilateral complete facetectomy, posterior ligaments resection, and the combination of the two resulted in an increase of the PJ angle (by 10, 28, and 53%, respectively), flexion forces (by 4, 12, and 22%, respectively), and proximal moments (by 16, 44, and 83%, respectively). Transverse process hooks at UIV allowed about 26% lower PJ angle and flexion loads. The use of proximal transition rods with proximal diameter reduced from 5.5 to 4 mm slightly reduced the PJ angle, flexion force, and moment (<8%). The increase in sagittal rod curvature from 10 to 40 degrees increased the PJ angle (from 6 to 19%), flexion force (from 3 to 10%), and moment (from 9 to 27%). Based on the simulation results, sagittal balance and proximal fusion level had significant effects on PJ angle, proximal bending moment, and proximal extensor force. A posteriorly situated T1 plumb line, as compared with an anteriorly situated one, was associated with higher PJ angle and proximal bending moment. Instrumenting more proximal vertebrae allowed lower PJ angle and proximal bending moment, thus lower mechanical risk of PJK. Avoiding posterior shift of the sagittal balance, reasonably extending instrumentation proximally, preserving PJ intervertebral elements, and using more flexible proximal anchorage each help reduce the biomechanical risks of PJK.
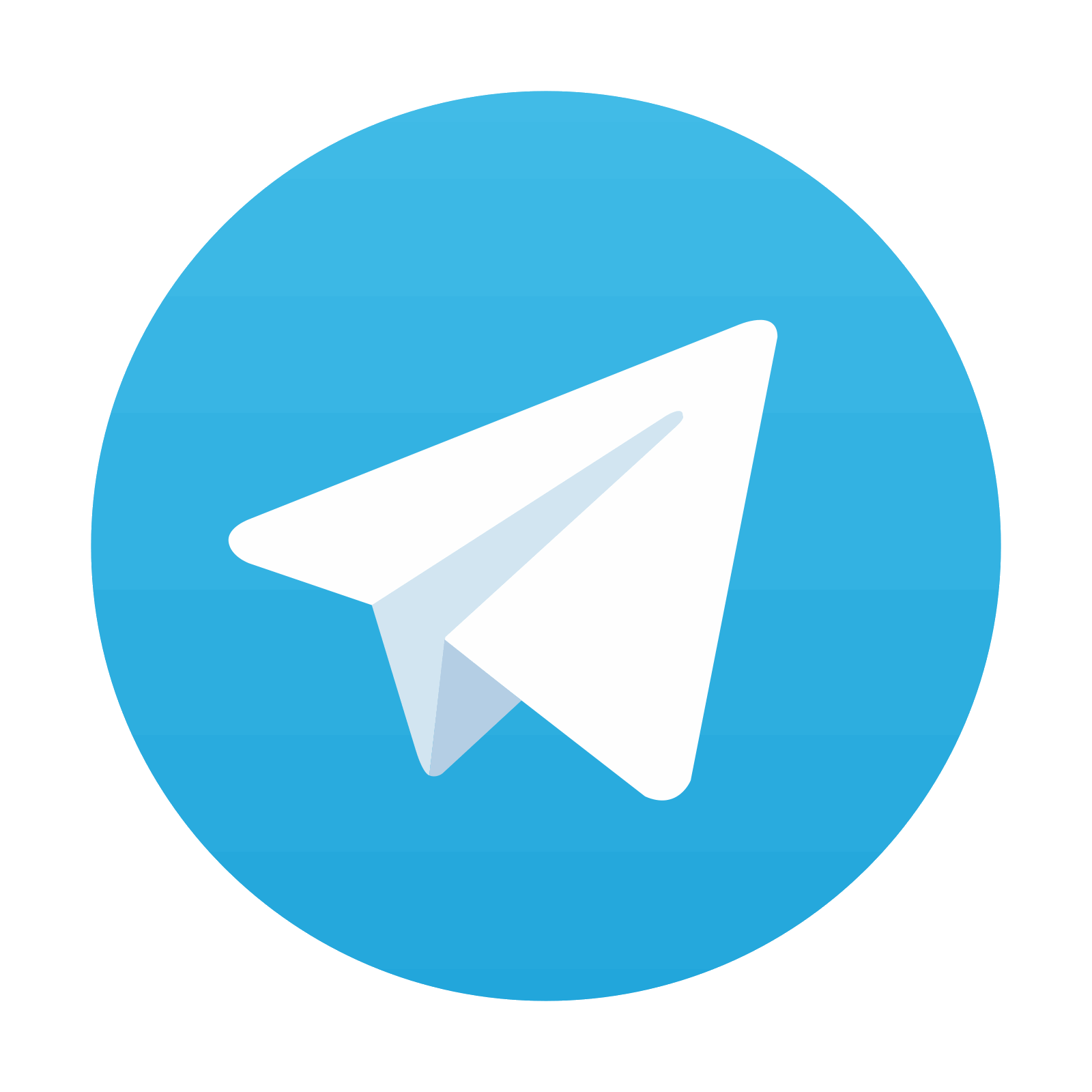
Stay updated, free articles. Join our Telegram channel
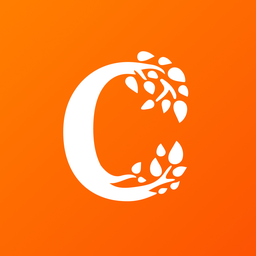
Full access? Get Clinical Tree
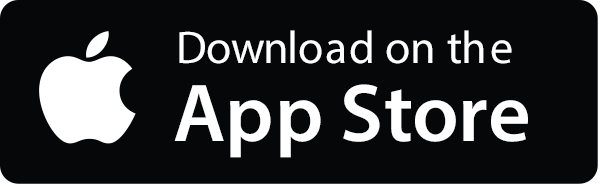
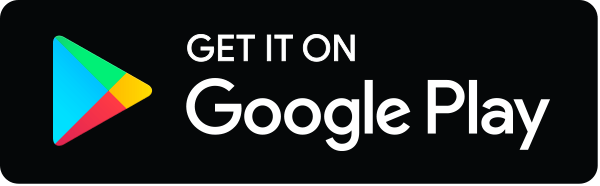