Fig. 11.1
ROS regulation of transcription factors and expression of proteins involved in promoting the reduction of fiber size due to disuse. Based on published findings, we propose a list of transcription factors along with E3 ligases and growth-arresting proteins known to directly promote atrophy or exert blunting effects on synthesis pathways, each of which is likely influenced by elevated myofiber ROS. This is a simplified figure illustrating ROS regulation of disuse fiber atrophy (refer to text for further discussion). Transcription factors listed do not necessarily correspond to the list of E3 ligases and growth-arresting factors. *Gadd45a and p21 are reportedly transcribed under the direction of ATF4 (Gadd45a & p21) and p53 (p21) [15, 19]
Altogether, a growing body of evidence suggests that oxidative stress can impede the rate of protein synthesis and accelerate protein degradation in a variety of ways, resulting in skeletal muscle atrophy. However, several gaps in knowledge are evident concerning ROS and disuse. Future studies should examine the specific mechanisms responsible for the rise in ROS production during disuse-induced skeletal muscle atrophy, as well as the notion of cross talk among oxidant sources. In the ROS-induced ROS-release model described by Andreas Daiber [12], activation of Nox and subsequent superoxide production could lead to depolarization of mitochondrial membrane ATP potassium channels. The reduction in mitochondrial membrane potential would then lead to increased mitochondrial-derived ROS, which could activate PKC. Active PKC has been shown to be capable of triggering Nox2 complex formation and activation. In this model, the initial ROS production from Nox2 signals for the augmentation and intensification of oxidative stress from the mitochondria [12]. In addition, activation of Fn14 has been shown to promote ROS production by stimulating Nox2 activity in macrophages [43]. Thus it is possible that Nox2 serves as an intermediate in an Fn14–Nox2–NFκB pathway. Clearly, there are many unanswered questions, and continuing research efforts will be required to elucidate our understanding of both the locations and regulation of ROS production during disuse-induced skeletal muscle atrophy.
11.5 Potential Role of Neuronal Nitric Oxide Synthase-mu During Disuse Atrophy
Currently, we do not have a full understanding of the complexities involved in oxidant production during disuse-induced skeletal muscle atrophy. Skeletal muscle wasting that occurs during pathological conditions, such as sepsis and chronic heart failure, has been associated with an overproduction of nitric oxide (NO) [2,55]. However, upregulation of iNOS, rather than nNOSμ, was cited as causal in cachectic muscle atrophy.
However, during disuse-induced atrophy, it is unclear whether excess NO production, source, or localization accelerates the atrophic process. In a study by Suzuki et al. [83], 14 days of hindlimb unloading was associated with a sarcoplasmic translocation of the mu (μ) – isoform of neuronal nitric oxide synthase (nNOSμ) from the sarcolemma-localized dystrophin glycoprotein complex (DGC) – and this translocation correlated with elevated levels of sarcoplasmic NO [83]. Their group followed this experiment up with genetic and pharmacological nNOS inhibition studies that indicated translocation of nNOSμ activity during hindlimb unloading as a critical event promoting atrophy [83]. Dystrophin remained attached to the DGC, while dysferlin, a multifunctional protein involved in membrane repair, remained bound to nNOSμ. Inhibition of nNOS prevented activation of FoxO3a and ubiquitin ligases [83]. Cytosolic levels of •NO were elevated as determined via EPR (electron paramagnetic resonance). We found that nNOSμ translocation is an early event in the mechanical unloading process, occurring within 3 days with the rat model [36]. In addition, Vitadello et al. [92] also found untethering of nNOSμ from the sarcolemma and translocation of nNOSμ with hindlimb unloading, linked to downregulation of grp94 [93]. Grp94 is an HSP90-like stress protein that chaperones protein folding [45]. Curcumin, the primary compound in the spice tumeric, with an antioxidant and anti-inflammatory properties mitigated nNOSμ translocation and atrophy [92].
In contrast, Lomonosova et al. [42] reported that L-arginine mitigated hindlimb unloading-induced reduction of nNOS, •NO, dystrophin, and HSP90 protein levels and mRNA transcripts gene expression. Heterogeneity in dystrophin sarcolemmal localization was found, suggesting disruption of the DGC. Furthermore, hindlimb unloading reduced levels of desmin [42], a Z-disc fiber protein that transmits loading to the DGC and surrounding extracellular matrix protein (e.g., laminin, collagen). Protein abundance was determined from serial frozen cross sections and thus assessed global levels, rather than site-specific changes. Efficacy of L-arginine may suggest a substrate limitation or decoupling of nNOSμ. In addition, a different spin trap was used for EPR in the Lomonosova study compared with Suzuki et al.: diethyl-dithiocarbamate (DETC) vs. N-methyl-D-glucamine-dithiocarbamate (MGD). Thus the global role of •NO and downstream nitrosative species remain uncertain in unloading-induced atrophy. However, we propose that (1) disruption of the DGC and membrane environment and untethering of nNOSμ from the sarcolemma, (2) subcellular location of nNOS and downstream effects on FoxO3a-ubiquitin ligase signaling, (3) substrate availability, (4) the role of other NOS isoforms, and (5) local •NO bioavailability may be more important than global •NO levels in regulating muscle fiber atrophy with mechanical unloading. However, additional research is crucial to unraveling these mysteries.
Interestingly, evidence indicates NOS and •NO are involved in the regulation of the type I MHC isoform [70, 82]. For instance, a recent report found that with 8 weeks of NOS inhibition, there was a significant decrease in the percentage of type I fibers and concomitant increase in the percentage of type IIa fibers in the soleus muscle [82]. Since disuse leads to a shift in fiber type from type I to type IIa, these data point toward the notion that a reduction in myofiber NO may be a critical event promoting the shift.
It is proposed that in healthy muscle, sarcolemma-localized nNOSμ is required for myofiber mechanotransduction. For example, muscle fiber hypertrophy with mechanical overloading was dependent upon intact sarcolemmal nNOSμ and NADPH oxidase 4 (Nox4) [27]. Genetic ablation or inhibition of nNOSμ early in the overloading process attenuated muscle hypertrophy by over 50% [27]. In support of these findings, the reduction in sarcolemma nNOSμ that occurs with unloading leaves the muscle fiber susceptible to sarcolemmal lysis by neutrophils upon reloading [54], thus slowing recovery, whereas mice expressing a muscle-specific nNOS transgene were significantly protected from sarcolemma damage and injury in response to reloading [54]. Clearly, nNOSμ plays a vital role as a load-sensitive molecule in skeletal muscle. Translocation of nNOSμ has been reported to occur in response to various forms of disuse: hindlimb unloading [36, 83, 90], spaceflight [66], denervation [83], bed rest [63], and intensive care-associated critical illness myopathy [40]. While some have observed disuse-induced alterations in nNOSμ synthesis and/or degradation, the mislocalization of nNOSμ in postural muscles is a common event associated with atrophy. Suzuki et al. [83] observed a causal link between nNOSμ untethering and proteolytic FoxO3a activation during hindlimb unloading. Our group demonstrated that administration of a superoxide dismutase (SOD)/catalase mimetic (EUK134) reduced the extent of nNOSμ untethering from the sarcolemma and translocation to the sarcoplasm following 54 h of hindlimb unloading [36]. This effect attenuated the activation of FoxO3a, subsequent atrophy, and shift in fiber type in the soleus muscle [36]. These data suggest that disuse-induced translocation of nNOSμ is due in part to a rise in oxidative stress.
Much remains to be learned concerning the many roles of nNOSμ during mechanical unloading. For example, further examination is needed to elucidate whether translocated nNOSμ leads to elevated localized NO within the sarcoplasm of the fibers and which signaling pathways are affected. Does nitrosylation of glutathione play a role in dephosphorylation of FoxO3a? What are the mechanisms of substrate limitation? What are the mechanisms underlying redox regulation of nNOSμ during unloading? A causal link between nNOSμ translocation that is directly associated with depressed protein synthesis is also of interest. In addition, identifying the molecular mechanisms guiding nNOSμ untethering from dystrophin and alpha-syntrophin in the DGC is of significant concern. In addition, are the effects of nNOS translocation fiber type dependent? Does nNOS translocation trigger fiber-type switch from slow to fast twitch with unloading?
11.6 Conclusions
In summary, disuse-induced skeletal muscle atrophy is due to elevation of ROS in the myofiber environment. Oxidative stress is implicated in the regulation of blunted rates of muscle protein synthesis and increased proteolysis (summarized in Fig. 11.2). Various myofiber oxidant sources participate in the production of ROS and are potentially involved in a ROS-induced ROS-release feedback mechanism. In addition, future studies are needed to determine the specific upstream signaling events that trigger the disuse-induced ROS production from each oxidant source. While the data remains unclear concerning NO production during disuse, there is a better understanding of the link between atrophying muscle and nNOSμ translocation. However, future studies are needed to elucidate the connections between oxidative stress, nNOSμ translocation, and the affected atrophic signaling cascades.


Fig. 11.2
Critical signaling pathways controlling muscle fiber size during disuse-induced atrophy and the regulatory schemes exerted by ROS. The combination of reactive oxygen species (ROS) derived from mitochondria, NADPH oxidase isoform 2 (Nox2), and xanthine oxidase (XO) results in an increased ROS concentration in myofibers during periods of disuse. Elevated ROS can blunt Akt-mTORC1 protein synthesis signaling, induce dislocation of nNOS from the DGC toward the sarcoplasm, enhance dephosphorylation of FoxO1/3a, and promote NFkB signaling. In addition, ROS from Nox2 and/or mitochondria may promote a ROS-induced ROS-release amplification scheme (as shown by the dashed red line with arrowheads). ROS regulatory functions are shown with red lines. Dashed lines indicate hypotheses concerning signaling events made by the authors based on published research findings. This is a simplified figure illustrating ROS regulation of disuse fiber atrophy; refer to text for brief discussions on alternative pro-degradation routes (e.g., calpains, caspase-3, autophagic–lysosomal)
References
1.
2.
3.
Allen DL, Linderman JK, Roy RR, Grindeland RE, Mukku V, Edgerton VR (1997) Growth hormone/IGF-I and/or resistive exercise maintains myonuclear number in hindlimb unweighted muscles. J Appl Physiol (1985) 83:1857–1861
4.
Arbogast S, Smith J, Matuszczak Y, Hardin BJ, Moylan JS, Smith JD, Ware J, Kennedy AR, Reid MB (2007) Bowman-Birk inhibitor concentrate prevents atrophy, weakness, and oxidative stress in soleus muscle of hindlimb-unloaded mice. J Appl Physiol (1985) 102:956–964CrossRef
5.
Awede B, Thissen J, Gailly P, Lebacq J (1999) Regulation of IGF-I, IGFBP-4 and IGFBP-5 gene expression by loading in mouse skeletal muscle. FEBS Lett 461:263–267
6.
Batt J, Bain J, Goncalves J, Michalski B, Plant P, Fahnestock M, Woodgett J (2006) Differential gene expression profiling of short and long term denervated muscle. FASEB journal: official publication of the Federation of American Societies for Experimental Biology 20:115–117
7.
8.
Bodine SC, Baehr LM (2014) Skeletal muscle atrophy and the E3 ubiquitin ligases MuRF1 and MAFbx/atrogin-1. Am J Phys Endocrinol Metab 307:E469–E484CrossRef
9.
10.
11.
12.
13.
de Boer MD, Selby A, Atherton P, Smith K, Seynnes OR, Maganaris CN, Maffulli N, Movin T, Narici MV, Rennie MJ (2007) The temporal responses of protein synthesis, gene expression and cell signalling in human quadriceps muscle and patellar tendon to disuse. J Physiol 585:241–251CrossRefPubMedPubMedCentral
14.
Derbre F, Ferrando B, Gomez-Cabrera MC, Sanchis-Gomar F, Martinez-Bello VE, Olaso-Gonzalez G, Diaz A, Gratas-Delamarche A, Cerda M, Vina J (2012) Inhibition of xanthine oxidase by allopurinol prevents skeletal muscle atrophy: role of p 38 MAPKinase and E3 ubiquitin ligases. PLoS One 7:e46668CrossRefPubMedPubMedCentral
15.
Ebert SM, Dyle MC, Kunkel SD, Bullard SA, Bongers KS, Fox DK, Dierdorff JM, Foster ED, Adams CM (2012) Stress-induced skeletal muscle Gadd45a expression reprograms myonuclei and causes muscle atrophy. J Biol Chem 287:27290–27301CrossRefPubMedPubMedCentral
16.
17.
Ferrando AA, Lane HW, Stuart CA, Davis-Street J, Wolfe RR (1996) Prolonged bed rest decreases skeletal muscle and whole body protein synthesis. Am J Phys 270:E627–E633
18.
19.
Fox DK, Ebert SM, Bongers KS, Dyle MC, Bullard SA, Dierdorff JM, Kunkel SD, Adams CM (2014) p 53 and ATF4 mediate distinct and additive pathways to skeletal muscle atrophy during limb immobilization. Am J Phys Endocrinol Metab 307:E245–E261CrossRef
20.
Glover EI, Yasuda N, Tarnopolsky MA, Abadi A, Phillips SM (2010) Little change in markers of protein breakdown and oxidative stress in humans in immobilization-induced skeletal muscle atrophy. Appl Physiol Nutr Metabol = Physiologie appliquee, nutrition et metabolisme 35:125–133CrossRef
21.
Goldberg AL, Etlinger JD, Goldspink DF, Jablecki C (1975) Mechanism of work-induced hypertrophy of skeletal muscle. Med Sci Sports 7:185–198PubMed
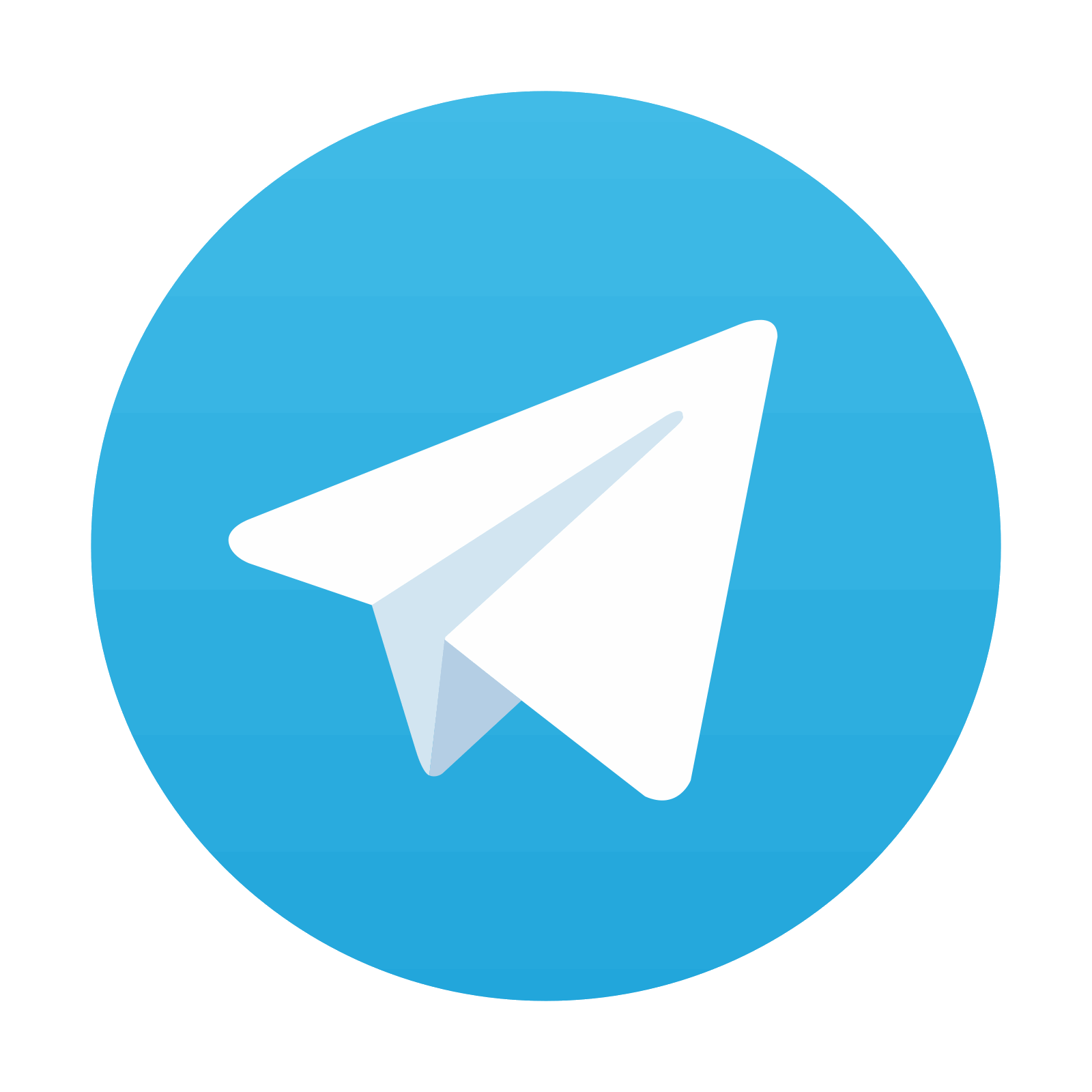
Stay updated, free articles. Join our Telegram channel
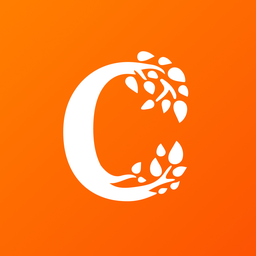
Full access? Get Clinical Tree
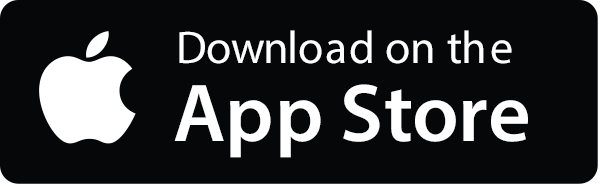
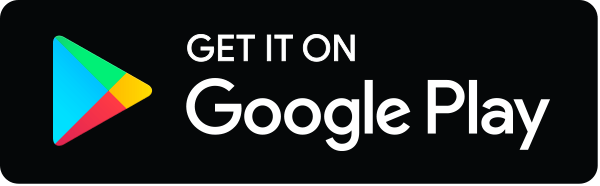