© ISAKOS 2017
Alberto Gobbi, João Espregueira-Mendes, John G. Lane and Mustafa Karahan (eds.)Bio-orthopaedics10.1007/978-3-662-54181-4_66. Physiology and Homeostasis of Musculoskeletal Structures, Injury Response, Healing Process, and Regenerative Medicine Approaches
Kaitlyn E. Whitney1, Ioanna Bolia1, Jorge Chahla1, Hajime Utsunomiya1, Thos A. Evans1, Matthew Provencher1, Peter J. Millett1, Robert F. LaPrade1, Marc J. Philippon1 and Johnny Huard1, 2
(1)
The Steadman Clinic and Steadman Philippon Research Institute, Vail, CO, USA
(2)
Department of Orthopedic Surgery, University of Texas Health Science Center at Houston, Houston, Texas, USA
6.1 Introduction
Treatments for musculoskeletal disorders have continued to progress by improved technologies and advancing a greater understanding of normal biology and biomechanics. Musculoskeletal biomechanical derangements may exist as a result of trauma, morphology, or chronic overuse. Biomechanical abnormalities can predispose the articular microenvironment to up-regulated inflammatory cytokines and may eventually lead to muscle atrophy and joint degeneration. Biomarkers can be used as predictors for diagnostic and prognostic strategies across diarthrodial joints that are at-risk for osteoarthritis (OA), but have yet to be instrumental in acute cases. Furthermore, the purpose of this book chapter is to comprehensively review physiological and homeostatic aspects, healing response and regenerative approaches for injured and surgically intervened connective tissues. This chapter describes physiological and homeostatic aspects of specific musculoskeletal structures of the knee, hip, and shoulder, the role of biomarkers in diagnostic and prognostic strategies, the healing response to surgical intervention and basic considerations for the use of regenerative treatment approaches for both conservative management and surgical augmentation.
6.2 Physiology and Homeostasis of Musculoskeletal Structures
6.2.1 Tendon
Tendons are of prime importance for stabilizing the knee, hip, shoulder, and other pivotal parts of the body that is comprised of vascular and avascular tissue. Tendon is composed of collagen type I, which is organized into microfibrils, fibrils, and fascicles surrounded by an endotenon sheath containing blood vessels, lymphatics, and nerves [1]. Tenocytes are arranged in parallel to the collagen fibril bundles and are responsible for turnover and maintaining the extra cellular matrix (ECM) [1]. It is uncommon for tendons to degenerate; however, when trauma or exessive overuse causes a tendon to rupture, surgical intervention is required to regain mechanical stabalization and promote vascular stimulation to the damaged tissue [3]. Tendinitis and Tendinosis are common overused injuries that are frequently viewed in athletes. Tendinitis and tendinosis typically respond to conservative care consisting of rest, nonsteroidal anti-inflammatory drugs (NSAIDs), and physical therapy, but in more severe cases may require surgical release or debridement [2].
Specifically, tendinitis is associated with inflammatory changes to the surrounding tendon sheath; tendinosis refers to degeneration of the tendon tissue itself and is characterized by disorganized collagen fibrils and increased tenocyte apoptosis, associated with increased risk of tendon failure [2, 4, 5]. It is understood that tendon rupture heals with an initial hemorrhage and inflammatory phase, involving cell migration to the site of injury and removal of tissue debris, followed by fibroblast infiltration, deposition of granulation tissue rich in collagen type III to form callus, and finally, remodeling of the collagen-rich scar tissue [6, 7]. Tissue repair mechanisms form scar tissue and express high levels of collagen type III, which weakens tissue durability and increases the risk of re-rupture [8]. Furthermore, adhesion formation can prevent normal tendon function and decrease joint motion. Biologics may serve as a conservative therapy for non-surgical conditions or surgical injection augmentation to promote stronger repair by enhancing collagen I production during scar formation.
6.2.2 Ligament
In a recent 10-year study, more than 6,500,000 patients with knee injuries in emergency departments accounted for a rate of 2.29 per 1000 people across all age groups [9]. Damage to ligaments of the knee is the most common form of knee injury [9]. Ligament and meniscal injuries cause instability and alter forces and loading patterns of the articular cartilage during movement [10]. Injuries to the intra-articular and extra-articular ligaments differ in regaining tissue homeostasis. Intra-articular ligaments have minimal to no ability to initiate tissue regeneration in response to injury. Surgical reconstruction is necessary to restore intra-articular ligaments anterior–posterior translational and rotational stability. In contrast, the extra-articular ligaments possess healing capabilities. The healing process begins by infiltration of pro-inflammatory cytokines and chemokines, followed by cellular infiltration, collagen deposition, and tissue remodeling [11]. Although lower grade ligament tears heal primarily with collagen I deposition, more severe tears are associated with collagen type III-rich scar tissue. Mechanical derangements of the knee are associated with biological changes of the synovial fluid and articular cartilage surface; this includes changes in collagen I and collagen III ratios [12], increased collagen type V [13], and changes in water and proteoglycan content [14]. High-grade extra-articular tears with bony avulsion or persistent laxity usually require surgical intervention with primary repair or reconstruction [11]. Treatment of high-grade tears may benefit from biologic therapies or tissue-engineering that express growth factors to improve the rate of repair and quality of repair tissue.
6.2.3 Bone
Skeletal tissue is the primary structural support for mechanical functions and produces intricate movements with connective tissue. Physiological loading of skeletal tissue activates anabolic pathways and stimulates osteogenesis [15]. Structurally, bone consists of two layers, the cortical bone and the trabecular bone. Within these layers, the strength and size of bone are determined by communication between osteoblasts, osteocytes, and osteoclasts cell derivatives [16] and biochemical signaling, such as the Notch signaling pathway, enables structural development and bone remodeling [17–19]. Other biochemical signaling pathways such as transforming growth factor-β (TGF-β) isoform and bone morphogenetic protein (BMP) isoform interactions initiate osteoblast cell lineages to activate and regulate bone formation [20, 21]. Although traumatic injury to the subchondral bone changes the biochemical profile, activating inflammatory and degenerative proteins that structurally alter and degenerate the trabecular bone [22].
Similar to articular cartilage, excessive training and overuse can cause mechanical derangements and lead to significant cartilage and osteochondral changes. The clinical manifestation of OA is described as the progressive deterioration of the articular surface and sclerosis of the subchondral bone. Moreover, the relationship between joint space and subchondral sclerosis has been identified in many joints [23–28]. Surgical treatment interventions are limited to resurfacing and debridement of the exposed subchondral defect [29]. However, numerous biological and gene therapy treatments used BMP and vascular endothelial growth factor (VEGF) have shown promising evidence by enhancing bone formation and vascularity [30–32]. Further analyses and clinical trials are warranted to elucidate its clinical impact.
6.2.4 Skeletal Muscle
Skeletal muscles are subject to direct (e.g., laceration, contusion, strain, avulsions) and indirect injuries (e.g., ischemia, infection, neurological dysfunction) [33]. Following injury, active muscle inflammation is generated during the first days as part of the healing tissue response [33], which is composed of three consecutive phases; muscle degeneration/inflammation, regeneration, and fibrosis [34]. Immediately post-injury, resident macrophages are activated and release various chemotactic molecules that attract neutrophils and monocytes to the site of injury. Tumor necrosis factor alpha (TNF-α) is the major coordinator of the initial degeneration and inflammation phase. While initially NSAIDS were thought to decrease pain and inflammation during this early stage, they have now been demonstrated to delay the proliferation and maturation of differentiated myogenic precursor cells [35], thus delaying the muscle regenerative process. Consequently, their effect on the muscle healing process remains controversial. The muscle regeneration phase begins during the first week post-injury and peaks at 2 weeks with a gradual decrease during the weeks 3 and 4. The major coordinators of this phase are the growth factor molecules, such as insulin growth factor 1 (IGF-1), basic fibroblastic growth factor (bFGF), and to a lesser extent nerve growth factor (NGF) [36]. Injecting human recombinant growth factor to the injury site constitutes a feasible way to reinforce the biologic processes of the second healing phase. Unfortunately, a very high concentration of these artificial proteins are required in order to achieve a desirable clinical outcome and consequently their use is limited. Finally, the fibrotic phase occurs, and the important role of TGF-β1 factor is implicated. This molecule is the major contributor to extracellular matrix production and deposition at the injured site, leading to fibrosis. Thus, research is now focusing on finding agents that decrease TGF-β1 production and/or block its action and effectively reduce the fibrotic response. In regard to muscle healing, platelet-rich plasma (PRP) combined with Losartan acts as an anti-fibrotic agent that neutralize the action of TGF-β1 and has been found to increase muscle repair and decrease fibrosis in mice models [37].
Moreover, the biologic treatments described above can be used as adjunct treatment to surgery and/or physical therapy to improve clinical outcomes. This is particularly important for elite athletes who seek a faster recovery and return to pre-injury level of performance.
6.2.5 Articular Cartilage and Fibrocartilage
Articular Cartilage. Articular cartilage serves as the primary tissue to enable gliding of articular surfacing in diarthrodial joints. Trauma, morphology, or chronic overuse can cause biomechanical derangements that are suggested to impact the articular surface of articular joints [38]. Excessive mechanical stress and trauma can lead to loss of articular surface regions and alter articular translation [38]. Damaged cartilage can form a defect, resulting in focal dissecans, OA, and in severe cases, collapse of the articular surface and subchondral bone. Gait and loading pattern abnormalities occur in consequence to the cartilage defect severity [39]. This is a specific morphology that causes bony displacement and subsequent pain, loss of function, and instability. The shoulder is another large joint that is prone to early development of OA due to capsular laxity and or labral lesions. Finally, associations between meniscal and ligamentous tears or injury of the knee and early OA have been observed. Changes in contact forces and mechanics of the joint lead to disruption of the ECM [8]. This in turn causes the release of glycosylated aminoglycans, collagen, and other connective tissue molecules that are sensed by surrounding cells. Biological imbalances occur when structural proteins alter gene expression in a cascade reaction, leading to the degeneration of articular cartilage [40]. There is little to no self-regenerating characteristics in cartilage due to the lack of vascularity, making this particular type of tissue difficult to repair, and having limited repair capacity [7]. Although, penetrating the subchondral bone to initiate bone marrow ventilation has considerable effectiveness through nutrient migration to the defect region [41, 42].
Standard procedures such as arthroscopic microfracture is commonly used to repair full-thickness cartilage defects by increasing type II collagen mRNA expression and ultimately promoting fibrocartilage scarring [43–48]. Several long-term significant outcomes following MF in younger patients have been reported [45, 46, 49, 50]. However, the integrity of the fibrocartilage scar tissue can be compromised while the subchondral bone regenerates new bone [51], and only 30–40% of the regenerated tissue is articular cartilage. Even though there are major challenges to repairing articular cartilage, these techniques have improved treatment of chondral and osteochondral defect. Several biological approaches are in development to advance alternative technologies and enhance cartilage regeneration.
Meniscus. The menisci are intra-articular structures with a medial crescent-shaped meniscus and lateral circular meniscus that are comprised of fibrocartilaginous tissue. The menisci attach centrally and peripherally to the joint capsule. Healthy menisci provide protection to the underlying articular cartilage to prevent excessive axial loading and degradation. In the presence of a cruciate ligament deficiency, the primary function of the menisci is to convert axial loading forces to minimize abnormal joint motion and force transmission. Trauma or overuse of the menisci can cause midline joint pain and swelling, alter load distribution, and predispose the articular cartilage to damage and OA [52]. Partial meniscectomy is frequently employed surgical interventions to remove damaged portions of the avascular meniscus and preserve meniscus function [53, 54]. It has been reported that surgical intervention improves knee range of motion and mechanical symptoms [54]. However, this technique does not address the underlying biological changes to the microenvironment that is attributable to OA [88]. Untreated meniscal tears may result in weight-bearing instability and disrupt mechanical loading [55–57]. Abnormal biomechanical patterns predispose the knee joint to early cartilage degeneration.
Biomechanical derangements caused by trauma, abnormal morphology, or chronic overuse can enhance the progression of tissue degeneration and have been suggested as a precursor of early OA [58]. In fact, OA can be characterized as a failed repair incurred by excessive mechanical stress, altered articular translation, and cellular and molecular changes to the articular environment [58]. These mechanical changes in the knee can eventually degrade the ECM, suppressing essential metabolic nutrients to the tissue [40]. In addition, the disruption of the ECM can cause a release of glycosaminoglycans, collagen, and other connective tissue molecules [40]. This, in return, causes imbalances of biomolecular properties that can alter gene expression, and ultimately lead to articular cartilage degeneration and fibrosis [40].
To further elaborate on the biological complexity, the inner two-thirds of the menisci lack adequate characteristics of self-repair [59]. However, the outer vascular portion of the meniscus consists of multilineage stem cells, while these cells are absent within the inner avascular region [59]. Native inner menisci fibrocartilaginous cells are attributable to outer meniscal healing through cell migration, proliferation, and differentiation in response to stimuli, but does not provide the robust healing to regenerate the inner meniscus [59–62]. High failure rate has been linked to repair of avascular meniscus tears due to the limited healing potential within the inner two-thirds of the meniscus, that often cause biomechanical alterations and articular cartilage degenerative and OA [57, 64, 65]. In this regard, biological therapy and tissue engineering may be useful alternatives to repair the avascular zone of the menisci.
Labrum—Hip. The hip labrum deepens the bony socket of the hip and is present around the entire lunate surface of the acetabulum. It is continuous with the transverse acetabular ligament inferiorly. The labrum extends the acetabular surface by 21% and maintains contact with the femoral head [66]. It enhances joint instability by providing structural resistance to lateral movement of the femoral head within the acetabulum, distributes forces, and provides a suction seal with the femoral head. This also is associated with synovial fluid management and maintainance of the cartilage integrity. Disruption of the suction seal mechanism predisposes to hip microinstability and early cartilage damage.
Injury to the chondolabral complex of the hip joint is relatively common. The fibrocartilaginous labrum detaches from the hyaline articular cartilage surface at the transition zone (chondolabral junction), which is usually 1-2 mm wide. Chondrolabral junction tears require less force for distraction and decreased femoral stability is seen during extreme ranges of motion [67, 68]. Labral tears also result in a significant increase in fluid efflux and decreased intra-articular fluid pressurization. The labrum has low permeability and the resistance of fluid flow is critical to joint sealing. The viscous dissipation in the normal labrum contributes to shock absorption, so poor fluid management by the torn labrum contributes to poor health in the hip joint.
Since 2005, most of the literature described labral debridement as the standard of care; however, as hip arthroscopy advanced, better outcomes were seen following labral repair [69]. Studies showed that the labral repair resulted in healing via fibrovascular repair tissue or direct reattachment with new bone formation [70]. In addition, research showed that the loss of labral tissue increased the negative effects of labral tears. Labral deficiency became more prevalent as hip arthroscopies increased and more revision hip arthroscopies were performed. This has resulted in labral tissue preservation in new labral treatments.
Surgical treatment of labral deficiency includes augmentation or reconstruction [71]. To improve healing in these procedures and for labral repair, biologics are commonly used. These have included PRP and bone marrow concentrate (BMC) [72]. While the literature is limited, these adjuncts have shown promising early results.
6.3 Injury Response, Healing Process, and Subsequent Diseases
6.3.1 Biomarkers
Acute Injury Biomarkers. Biomarkers are categorized according to the five categories of the “BIPED” classification proposed by the National Institutes of Health (NIH)-funded OA Biomarkers Network which includes five categories: Burden of Disease, Investigative, Prognostic, Efficacy of Intervention, and Diagnostic (BIPED) [73]. The BIPED classification system is becoming a useful tool in diagnostic and prognostic strategies for patients affected by tissue degeneration and OA. The current classification system for other damaged tissues, such as muscles, ligaments, and tendon, has historically been based on enzymes in serum and plasma, for example, creatine kinase, aldolase, and lactate dehydrogenase [74, 75]. Other biomolecular methods have been reported to establish a profile for muscle, ligament, and tendon injuries but are not well adopted in sports medicine and orthopedic diagnostic strategies [76, 77].
Chronic Injury Biomarkers. There is a strong correlation between clinical status and disease pathology, yet no single biomarker or a combination thereof has been reported to possess adequate sensitivity or specificity for clinical use [78, 79]. Biomechanical imbalances within various tissues may exist as a result of trauma, morphologic abnormalities, or chronic overuse. These conditions may produce an up-regulation of inflammatory cytokines that can act as primary stimulators that are indicative of symptomatology and the progression of tissue degradation [80, 81].
Biomarkers and their corresponding gold standard concentrations can be used as a tool for diagnostic and prognostic skills. In doing so, biological or physiological derangements shared by subgroups will be recommended a specific treatment that are tailored to biomarker results [82]. Burden of disease assesses biomarkers that are linked to the severity or extent of disease at a single point in time (e.g., serum cartilage oligomeric matrix protein (COMP) [83], serum hyaluronan [84], and urinary CTX-II) [85]; the investigative category classifies biomarkers with limited information on trademark effectiveness or efficacy in reducing detrimental biomarker concentrations (e.g., collagen type II cleavage [C2C]); the prognostic category is classified as the ability to predict future onset of the disease or disease activity along with the recommendation of certain beneficial treatment modalities (cluster of differentiation [CD] 163, type I collagen degradation biomarker [C1M]); efficacy of intervention assesses the efficacy of a certain treatment in patients with the disease or at-risk (IL-10, Fib3-1); lastly, the diagnostic category classifies individuals with various musculoskeletal pathologies depending on indicative biomarker levels (e.g., brain-derived neurotrophic factor [BNDF]). These tests should be evaluated and compared in a gold standard setting with an appropriate spectrum of subjects [73]. Kraus et al. suggested the addition of an “S” (for safety) to the BIPED classification. The “S” would classify the monitored health status of various tissues or general cytotoxic status in response to treatment [86].
Additionally, there are tissue-specific biomarkers that are indicative of cartilage degradation (e.g., carboxy-terminal telepeptides of type II collagen [CTX-II], collagen type I [C1], collagen type II [2C], C2C, peptide form and nitrated form [Coll2-1NO2], cartilage oligomeric matrix protein [COMP], C-propeptide CPII, type-IIA collagen N-propeptide [PIIANP], chondroitin sulfate epitope 846 [CS846], matrix metallopeptidase-3 [MMP-3]), bone resorption (serum and urine N-terminal telopeptide of type I collagen [NTX-I] and C-terminal telopeptide of type I collagen [CTX-I]), and synovitis (serum hyaluronic acid [HA]) [87]. The characterization of biomarkers needs to be assessed further in general populations with a musculoskeletal condition.
6.3.2 Osteoarthritis
In the hip, shoulder, and knee, biomechanical derangements may exist as a result of trauma, morphology, or chronic overuse. Biomechanical abnormalities can predispose the articular microenvironment to an up-regulation of inflammatory cytokines, but there is little supporting evidence of this process occurring in overall clinical applications. Contemporary literature suggests that certain pro-inflammatory cytokines are predictors and primary stimulators for symptomatology, cartilage breakdown, and degeneration [80, 81]. Loss of the articular cartilage lining the load-bearing surface of bone (joint space narrowing), osteophyte formation, subchondral sclerosis, subchondral cysts, bone marrow lesions, at times synovial proliferation and synovitis, degeneration of intra-articular soft tissues, and often muscle atrophy are common indications of OA that are observed by radiographic and magnetic resonance imaging (MRI) [89, 90]. OA is a multifactorial disease that progressively deteriorates tissue with several risk factors involved, such as trauma and excessive overuse, gender, genetic factors, obesity, and aging [91, 92]. Additionally, mechanical dearrangements occur, causing biological imbalances and degradation of the articular surface and subchondral bone. Common clinical symptoms that arise include decreased range of motion, crepitus, deformity, joint instability, and joint dysfunction.
The pathophysiology of OA begins with damage to the collagen structure [89, 90, 93, 94], degenerative growth factor stimulation and infiltration [95–98], dramatic changes to the cartilage ECM [99], and chondrocyte apoptosis [100]. Subsequent to mechanical injury, collagen degradation also occurs [101]. In a healthy diathrodial joint, collagen type II is inherently responsible for new collagen fibril formation [102]. The degradation proteins that provide structure to the ECM, such as collagen type II, collagen XI, collagen IX, collagen XII, collagen VI, COMP, matrilin 1, matrilin 3, fibrillins, perlecan, fibromodulin, versican, decorin, lumican, mimecan, epiphycan, fibronectin, thrombospondins, tenascin C, CILP, link protein, biglycan, and nidogen, causes further tissue degeneration [99].
Similar to chondral and osteochondral defects, repair and treatment of early and end stage OA are limited, and usually necessitates total joint arthroplasty. OA may be treated with conservative management that implies a lifestyle change of diet, exercise, and overall health, but can also be treated nonoperatively with interventional pain management strategies [103, 104]. Although these treatments may delay the need for joint replacement, considerations for biological therapeutics are warranted to delay the progressive deterioration of articular tissue in early OA patients and improve functional ability of patients with end stage OA.
6.4 Regenerative Medicine Approaches
6.4.1 Autologous Biologic Therapies
Platelet-Rich Plasma. Platelets are ubiquitous blood components that are made of dense material called α-granules that are capable of storing and releasing proteins [105]. Platelets provide homeostatic, coagulatory, pro-inflammatory, and anti-inflammatory roles by activating α-granule-specific growth factors and other proteins [105]. PRP is made up of multifaceted bioactive properties that demonstrate cellular adhesion and regenerative capabilities. Other proteins such as fibronectin, vitronectin, fibrinogen, prothrombin, IGF-1, and hepatocyte growth factor (HGF) naturally reside in plasma and are also emitted from α-granules upon platelet activation [105]. Certain growth factors are selectively regulated by granule proteins for either growth factor activation or inhibition [106].
Additionally, PRP can create a pro- or anti-inflammatory environment by secreting chemokine and specific interleukins. Pro-inflammatory factors are important signalers and activators that induce cellular responses. High concentrations of pro-inflammatory factors can create an imbalance within the environment and inhibit other signaling pathways. PRP stimulates anti-inflammatory mechanisms to balance pro-inflammatory factors and reduce subsequent inflammatory responses [107, 108]. There is an ongoing debate regarding pro-inflammatory factor utilization to enable surrounding healing mechanisms. Leukocyte-rich PRP has been reported to increase cellularity and new vasculature in an acute inflammatory environment in tendinopathy cases [109]. In contrast, leukocyte poor PRP has been reported to be a better treatment modality for intra-articular pathology since it is believed to induce greater cell growth by stimulating chondrocyte anabolism, whereas leucocyte-rich PRP promotes catabolic pathways involving various cytokines [110] and can produce significant side effects [111]. Studies suggest that PRP is capable of reducing pain and improving functional status, especially in patients affected by early to moderate OA [112].
Bone Marrow Concentrate. Bone marrow concentrate (BMC) treatment for bone healing has been shown to promote osteoinduction and enhance bony surface bridging [113–115]. Contemporary literature suggests that BMC augmented in surgical intervention of large cartilage and osteochondral lesions can improve regenerative potential and regenerate hyaline-like cartilage [116–120]. Kim et al. demonstrated the conservative utility of BMC treatment in knee OA and reported improvements in visual analogue pain scores by an average of three points [121]. Patients also reported a 31.6 average improvement from baseline scores in International Knee Documentation Committee scores at 12 months post-procedure [121]. There are many clinical observations of BMC therapy for the treatment of knee OA that have resulted in positive clinical outcomes. Like many cell-based therapies, there are inconsistencies in application [122] and clinical outcomes [123]. This may be due to inhibition of platelet-derived anti-inflammatory factors. In addition, the number of bone marrow-derived mesenchymal stem cells varies depending on the location of harvest, gender, and patient age, but overall the concentration of mesenchymal stem cells constitutes only a small portion of the bone marrow. In current efforts to optimize cell-based therapies, mesenchymal stem cells and chondrocytes are frequently used for cartilage and bone regeneration. BMC is not only composed of multiple types of progenitor stem cells, it is also composed of leukocytes, erythrocytes, and platelets. Platelets in BMC are capable of activating and releasing hundreds of biologic markers, such as growth factors, interleukins, cytokines, and chemokines. Fortier and colleagues were the first to examine platelet constituents and measure bone marrow-derived mesenchymal stem cell markers in BMC and compare concentrations to PRP between two separate processing systems [124]. BMC was reported to have greater growth factor concentrations and a significantly greater interleukin-1 receptor antagonist (IL-1RA) concentration [124]. IL-1RA (inhibits IL-1 catabolism) is thought to be responsible for the beneficial effects of autologous conditioned serum and reducing cytokine pro-inflammatory stimulation [124]. The number of progenitor stem cells, specifically mesenchymal stem cells, varies depending on the location of harvest, gender, and patient age, but overall the concentration of mesenchymal stem cells constitutes only a small percentage of mesenchymal stem cells present. Previous literature has suggested that the therapeutic effects of mesenchymal stem cells may be mediated by secreted factors [125, 126]. Growth factors are secreted from platelet α-granules that naturally reside in peripheral blood and bone marrow. Growth factors are able to mitigate mesenchymal stem cell transformation into various types of cell lineages while normalizing the inflamed microenvironment.
Several studies have demonstrated the advantages of isolated bone marrow-derived stem cell application for musculoskeletal tissue repair and regeneration by demonstrating excellent results in cell proliferation and differentiation [127, 128]. Bone marrow-derived mesenchymal stem cells are multipotent cells that are harvested and isolated from bone marrow. Even though bone marrow serves as a reservoir of secreting molecules, the necessary dose of bone marrow-derived mesenchymal stem cells drawn from autologous or allogenic bone marrow is inadequate for tissue regeneration. A consensus has not been established on whether patient demographics (e.g., gender, age, and other patient demographics) influence the production of bone marrow-derived mesenchymal stem cells. Further analyses are necessary to characterize, customize, and optimize the clinical application of BMC and enhance patient outcomes.
6.4.2 Gene Therapy
Gene therapy is the process of delivering biological factors (e.g., growth factors, lubricin enzymes) and/or cells (e.g., muscle-derived stem cells, mesenchymal stem cells, hematopoietic stem cells) to the intracellular zone of the tissue to alter transcriptional profile in damaged musculoskeletal tissue [129]. This process enables production of therapeutic morphogens, growth factors, and anti-inflammatory factors via gene expression [129]. Moreover, this therapeutic approach aims to enhance genetic expression and promote tissue healing and restoration.
There are two ways to administer gene therapy: (1) in vivo delivery is administered peripherally. This gene-based approach is prepared as a vector transgene construct [129]. And, (2) ex vivo delivery is directly applied to the lesion site following stem cell isolation, proliferation, passage, and then prepared by non-viral transfection [129].
Superior healing and regenerative characteristics have been observed in the ligament [130, 131], tendon [132, 133], muscle [134, 135], fibrocartilage [136, 137], and focal cartilage and osteochondral defects [138, 139]. Treating large cartilage and osteochondral defects, and OA using gene-based therapies, remains challenging given the surface area of damaged tissue. Although, combinational gene therapies and growth factor-based therapies are emerging alternatives to treat larger defects and disease [140].
Therapeutic proteins have provided efficacious outcomes when primary role of growth factors (e.g., VEGF, PDGF, TGF-β, FGF, and IGF) is to initiate and/or inhibit cellular signaling pathways. Although, microenvironmental factors, such as pH, drug carrier, mechanical stimuli, and serum can influence the delivery and overall course of the growth factors [141].
Delivery methods are recapitulating to increase growth factor retention to initiate intracellular signaling and tissue formation. Despite the advancements in gene therapy approaches, several barriers exist that hinder the clinical translation of this technology [129].
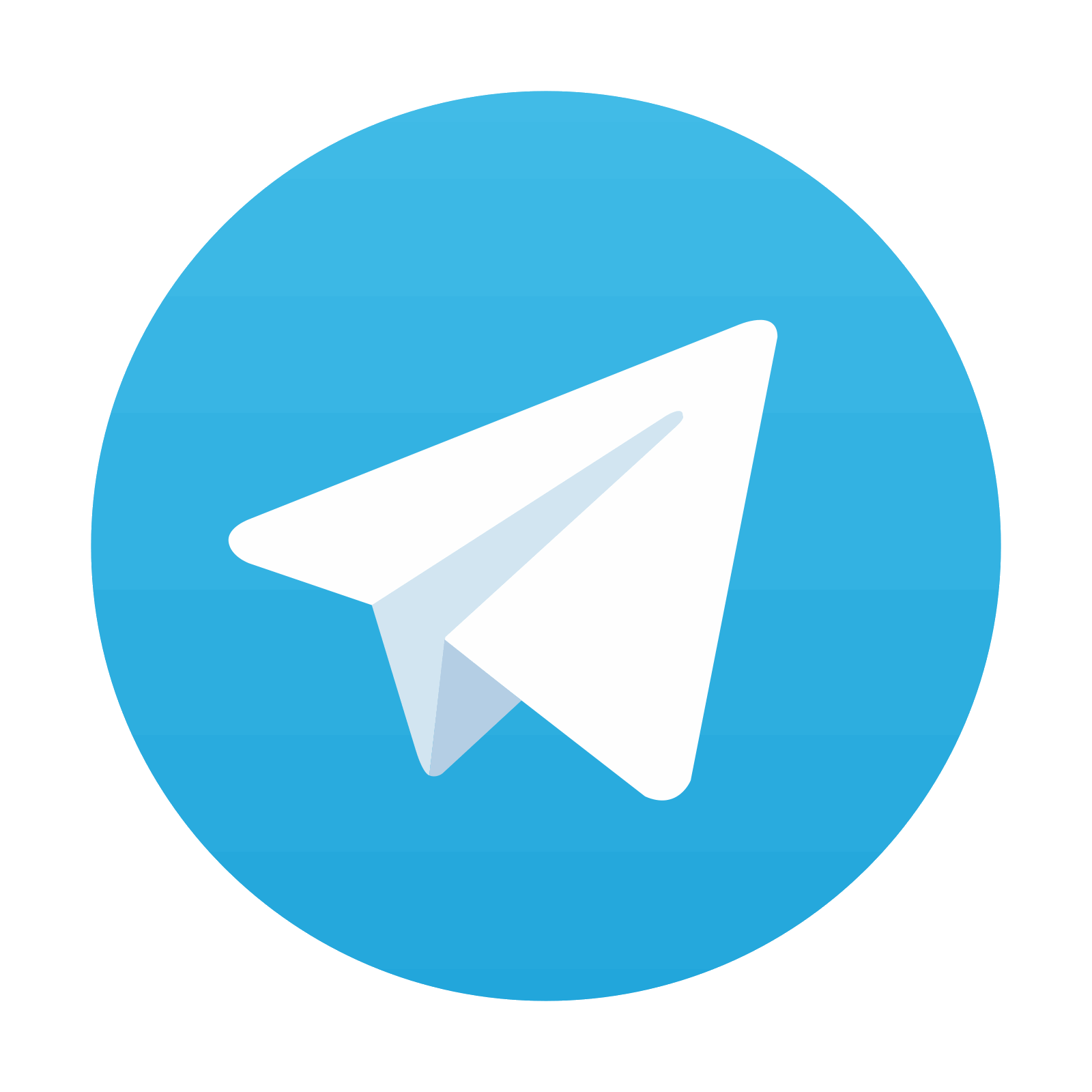
Stay updated, free articles. Join our Telegram channel
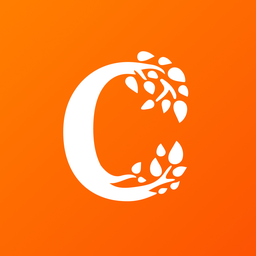
Full access? Get Clinical Tree
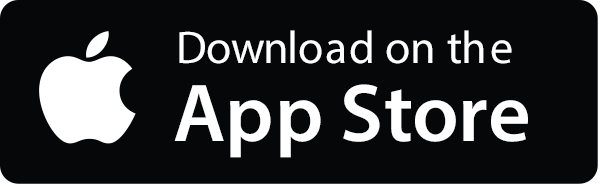
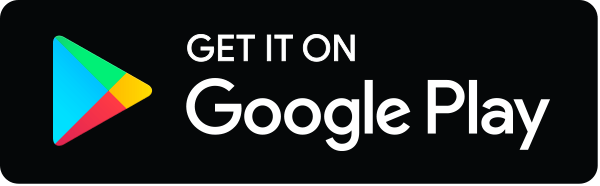