Vascular region
Blood flow (ml.min−1)
Rest
VO2max
Brain
750
850
Coronary
250
1000
Kidneys
1200
360
Muscle – Inactive
1000
850
Muscle – Active
21,840
Skin
500
300
Splanchnic
1500
350
Other
300
100
Total
5500
25,000
3.2.2 Skeletal Muscle Heat Production
Active muscles are a major site of endogenous heat production and storage. Indeed, exercise performed at 80–90 % VO2max could increase core temperature by 1 °C every 5–8 min if heat was not dissipated to the environment. This is due to the low mechanical efficiency of humans, as approximately 70–75 % of the energy derived from metabolism during exercise is released as heat, rather than converted into movement [24]. However, the circulation of blood ensures temperature regulation, which is an essential physiological function that controls the exchange of heat between the different human body segments and the environment.
At the onset of exercise, heat production in active muscles increases sharply and markedly from a resting temperature of about 34 °C. During short intense efforts the production of heat increases until the termination of exercise. For example, during a 3 min maximal effort of the knee extensors, heat production is doubled, with 50 % of the increase occurring in the first minute [26]. In contrast, the rate of rise in muscle temperature is curtailed and production eventually plateaus during steady state dynamic exercise. Nevertheless, the absolute increase in skeletal muscle temperature when transitioning from rest to prolonged intense dynamic exercise can exceed 5 °C [30]. The increased heat production is linked to both anaerobic and aerobic energy pathways. The initial rise in temperature is associated with an anaerobic energy turnover, until a shift to aerobic metabolism occurs (i.e. glycogenolysis). Interestingly, exercise performed under heat stress has been shown to enhance glycogen utilization in the contracting musculature [17, 18]. However, the development of skeletal muscle fatigue during prolonged exercise in the heat appears to be unrelated to substrate availability [16].
Rather, exercise in the heat leads to a significant amount of blood being redirected towards the skin for the purpose of heat dissipation. Although this redistribution does not compromise muscle blood flow during submaximal exercise [35, 54], it does exacerbate the cardiovascular response [51]. As a result, VO2max is reduced during protracted exercise in the heat, which leads to an increase in relative exercise intensity for any given workload [4, 39, 64]. Ultimately, the increase in intensity results in the early cessation of constant rate exercise, or the reduction of power output/running speed during self-paced efforts. At the level of the muscle, the combination of an accumulation of heat and prolonged contractile activity have been shown to compromise cellular and neuromuscular function, which are likely to impair performance [25, 41, 59].
3.3 Skeletal Muscle Fatigue and Heat Stress
A moderate increase in skeletal muscle temperature enhances brief explosive performance (e.g. sprinting and jumping) by improving metabolic function, nerve conduction and conformational changes associated with muscle contraction [2, 12, 20]. Muscle contractile function is also improved in a heated muscle, as demonstrated by increases in the rates of force development and relaxation (Fig. 3.1). Although these increases benefit short-term power output and force development, they require faster motor neuron firing rates to maintain the fusion of force during prolonged efforts (e.g. maximal voluntary isometric contractions). Thus, a sustained contraction performed at a given absolute force with a heated muscle will result in a more rapid decline in force, compared with a cooler muscle, as firing rates decrease [12].


Fig. 3.1
Muscle contractile response to a single brief threshold stimulus (i.e. twitch) in normothermic (blue) and hyperthermic (red) skeletal muscle. A twitch has three phases: a latent period, a period of contraction, and a period of relaxation. From this response, peak twitch force, time to peak force, half-relaxation time, along with rates of force development and relaxation may be calculated to evaluate the changes in the contractile function induced by exercise or heat stress
Comparative studies of mammalian muscle contractions in vitro and performance in vivo have also indicated that muscle fatigue is temperature-sensitive. It appears that optimal muscle temperature for performance, the temperature at which less fatigue develops, is achieved around 30 °C [48, 58]. However, these results may not relate directly to exercising humans, as resting core and muscle temperature are typically 7 °C and 4 °C higher, respectively. Nevertheless, in humans performing whole-body dynamic exercise there is a dose-response relationship between temperature and performance [47]. This relationship is non-linear and dependent on central (i.e. core) and local (i.e. muscle) temperatures, whereby it is a rise in muscle temperature that improves power output [14] and a rise in core temperature that leads to impairment [47]. For example, mean power output during a repeated sprint protocol on a cycle ergometer was improved in hot ambient conditions with the attainment of a core temperature of ~38.1 °C, versus ~37.7 °C in temperate conditions [23]. Conversely, repeated sprinting ability was reduced when core reached ~39.5 °C in the heat, compared with ~38 °C in cool conditions [10].
Part of the loss in performance associated with the attainment of an elevated core temperature has been attributed to a hyperthermia-induced reduction in central neural drive to exercising muscles (i.e. central fatigue) [32, 37]. Indeed, several studies have shown that passive hyperthermia is associated with a loss of strength, concomitant with reductions in voluntary activation [32, 40, 41, 45, 60]. However, the role of exercise-induced hyperthermia in exacerbating central fatigue remains unclear. It was previously shown that voluntary activation and force production progressively decrease during a sustained (120 s) maximal voluntary isometric contraction following exhaustive cycling in the heat, but remain elevated after exercise in cool conditions [37]. Conversely, more recent studies have reported that voluntary activation and strength losses are equivalent during brief (5 s) and sustained (20 s) contractions conducted after self-paced and incremental exercise in hot and cool conditions [42, 46]. Part of the discrepancy may relate to the length of contraction. After 30 s of maximal isometric effort pain becomes increasingly severe, altering the perception of sensations originating from the active musculature, which leads to uncertainty as to the level of force being exerted [5]. Moreover, a lack of motivation during prolonged contractions might reduce central activation to the appropriate motor neurons, resulting in the loss of force [13].
During exercise in the heat, fatigue is also mediated by adjustments occurring beyond the neuromuscular junction (i.e. peripheral fatigue) [40–42, 45]. These peripheral adjustments contribute to the preferential development of fatigue at low frequencies of stimulation (i.e. low-frequency fatigue) [11]. Consequently, force production in hot fatigued muscles decreases at lower stimulation frequencies, shifting the force-frequency relationship toward higher frequencies (Fig. 3.2) [43]. This phenomenon has been attributed to a failure in excitation–contraction coupling, stemming from a reduction in sarcoplasmic reticulum Ca2+ release [11, 27, 63], and/or a conduction block of the action potential [2, 20]. It has also been linked to a reactive oxygen species mediated decrease in myofibrillar Ca2+ sensitivity [7]. Interestingly, the influence of the failure in excitation–contraction coupling is negligible at high frequencies of stimulation, as the total number of action potentials increases. As a result, force production in hot fatigued muscles decreases at lower excitation frequencies, but remains elevated or only slightly decreases at higher frequencies.


Fig. 3.2
Force production capacity relative to maximum force output in normothermic-unfatigued (blue), hyperthermic-unfatigued (orange) and hyperthermic-fatigued (red) skeletal muscle. A rightward displacement of the force-frequency relationship occurs at lower excitation frequencies due to the development of hyperthermia and low-frequency-fatigue. In hyperthermic-fatigued muscle, a greater excitation frequency is required to attain 50 % of maximal force generation, an effect that diminishes as frequency increases. Adapted from Périard et al. [43]
3.4 Exercise-Induced Muscle Damage
Muscle damage stemming from physical activity occurs following unfamiliar forms of exercise or extended periods of intense exercise. Interestingly, it appears that muscle damage and the recovery of functional performance are not aggravated following strenuous exercise under heat stress, compared with exercise undertaken in temperate conditions [36]. On the other hand, movements that involve eccentric muscle loading or lengthening contractions tend to exacerbate the damage and increase the risk of injury [8, 44]. Fast-twitch muscle fibers are especially susceptible to eccentric damage. This is likely due to structural differences between fiber phenotypes, as well as the greater level of generated tension and lower oxidative capacity of fast-twitch fibers [44]. Characteristically, exercise-induced muscle damage causes an immediate loss of strength, which can take a number of days to recover (Fig. 3.3). Swelling and soreness within the muscle develop thereafter (1 or 2 days), in conjunction with the release of soluble muscle proteins such as creatine kinase. Acute and chronic inflammatory responses are also associated with damaging exercise.


Fig. 3.3
Time course of adjustments in skeletal muscle damage following maximal eccentric exercise. Force loss, muscle soreness, creatine kinase activity, and inflammation (acute and chronic) are plotted on a relative 10-point scale (0 = no increase, 10 = large increase) of arbitrary units (AU) (Adapted from Clarkson and Hubal [8])
The precise mechanism that induces muscle damage is not fully understood. The initial trauma appears to originate from mechanical disruptions to actomyosin bonds within the muscle fibers. It is suggested that stretching the muscle beyond 140 % of its optimal length results in unfamiliar strain to the fibers [15]. This causes a linear deformation to certain sarcomeres within myofibrils and leads to membrane deformation, particularly in T-tubules, as well as disruption to calcium homeostasis. Consequently, damage occurs in response to the tearing of membranes and opening of stretch activated channels [1, 15]. Following the initial trauma, alterations in excitation-contraction coupling occur in conjunction with the inflammatory processes, which are characterized by infiltration of fluid and plasma proteins into the site of trauma [8]. The extent of the muscle damage is dose-dependent, as greater volumes of exercise produce greater trauma. Interestingly, the magnitude of trauma during a subsequent bout of similar damaging exercise is reduced relative to the first bout [31]. This phenomenon is called the ‘repeated bout effect’ and can last for several months [56]. It is proposed that this adaptation stems from a strengthening of the connective tissue, a greater efficiency in motor unit recruitment and synchronization, a better distribution of workload amongst fibers, and a greater contribution of synergistic muscles during work.
In humans, the direct assessment of muscle damage is difficult. It requires the analysis of muscle biopsies, or the interpretation of magnetic resonance imaging (MRI). Although biopsies provide information about myofribrillar disturbances and Z-line streaming, the characterization is specific to a certain portion of muscle. As such, biopsies may not represent the state of the entire muscle [8]. Conversely, MRI assesses damage through the edemic response in the whole muscle by using signal intensity changes (i.e. T2 relaxation time). This technique is naturally better tolerated, as it is non-invasive. However, MRI interpretation remains problematic, as it may not be clear what the changes in the images indicate. As such, indirect markers of muscle damage are often used to evaluate the level of trauma associated with damaging exercise. These markers offer strong insight into the level of damage. The three indirect markers most commonly used are subjective soreness, blood proteins, and maximal voluntary force production [62].
3.4.1 Muscle Soreness
Following a bout of damaging exercise, muscle soreness does not appear for several hours, peaking at 24–48 h after exercise. This response is called delayed onset muscle soreness (DOMS). The level of soreness is associated with the level of muscle damage incurred, which is typically characterized by the type of activity performed. For example, non-weight bearing exercise such as cycling, which produces very little muscle damage, will result in minimal soreness. In contrast, maximal repeated eccentric contractions of the knee extensors will produce significant levels of muscle soreness. Part of the soreness is derived from swelling and pressure within the muscle. As fibers become enlarged, intramuscular pressure rises to cause pain [21]. The sensation of soreness also stems from the release of noxious chemicals (e.g. histamines, prostaglandins). These activate type III and IV muscle afferents, which trigger the sensation of pain [38]. However, muscle nociceptors can become sensitized to these noxious chemical mediators. In such circumstances, the mechanical deformation and swelling of the fibers act as the main physical stimulus for pain/soreness [28]. Interestingly, peak levels of soreness occur long before peak swelling.
3.4.2 Creatine Kinase and Myoglobin Activity
Hematologically, several muscle enzymes (e.g. lactate dehydrogenase, aspartate aminotransferase) and proteins (e.g. myoglobin, troponin) can be measured following exercise to indirectly assess muscle damage. Due to its significant magnitude of increase relative to other proteins, creatine kinase (CK) in the bloodstream has been utilized most often as a blood marker. Depending on the mode and intensity of exercise, CK values can peak between 12–24 h (e.g. downhill running), or 4–6 days (e.g. maximal eccentric contractions) [9, 57]. A particular limitation with using CK however, is the large variation in response among individuals [8]. Notwithstanding, CK activity in the blood is generally associated with the level of damage assessed via MRI. It therefore represents an indirect relative marker of muscle damage. Similarly, when muscle fibers are damaged, myoglobin in the cells is released into the bloodstream (i.e. rhabdomyolysis). The presence of myoglobin in urine and serum is indicative of skeletal muscle trauma, inflammation and ischemia. In extreme circumstances it may indicate a heart attack, or malignant hyperthermia. As such, myoglobinuria represents a useful marker of muscle damage during exercise [36], especially in hot environmental conditions since hyperthermia may aggravate rhabdomyolysis [33].
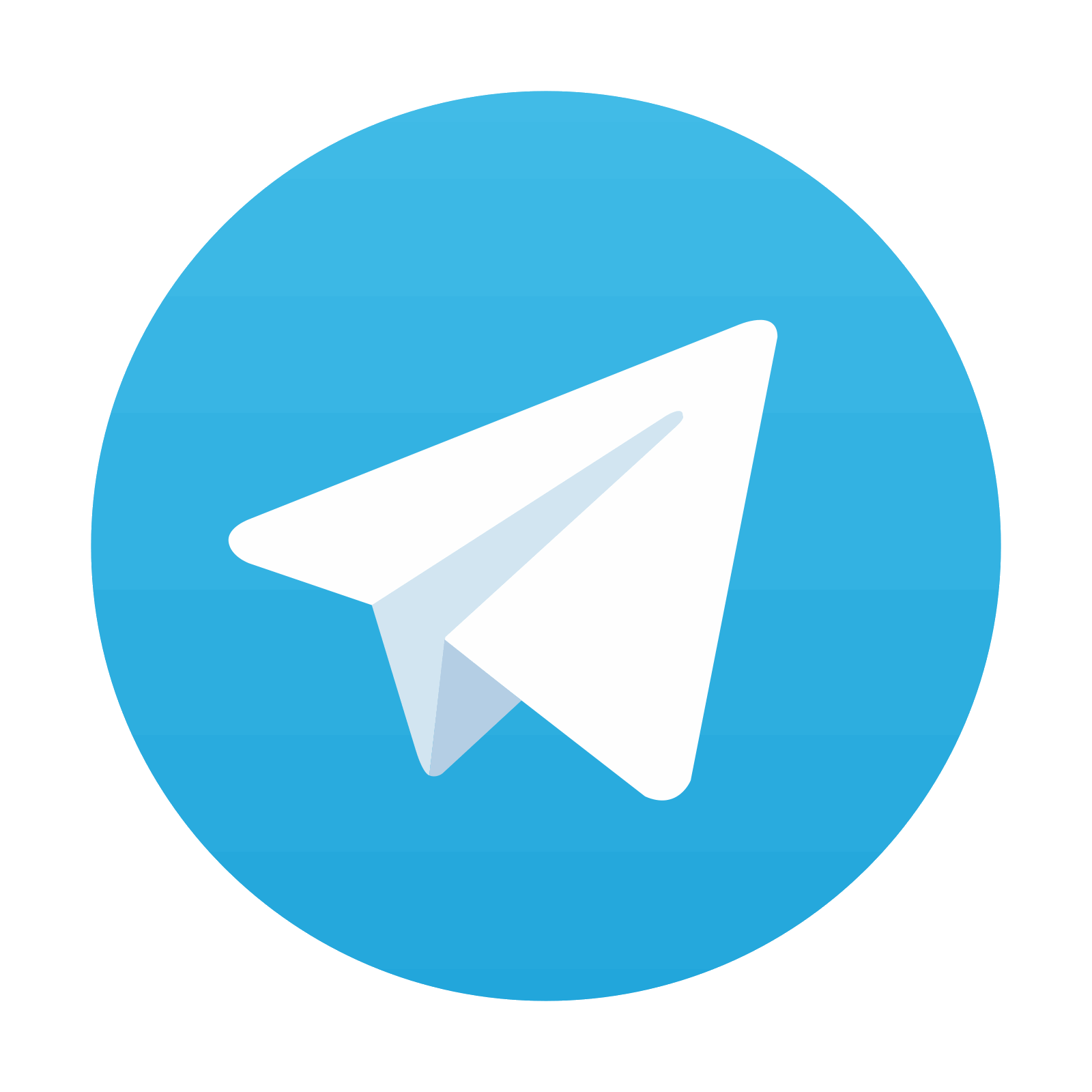
Stay updated, free articles. Join our Telegram channel
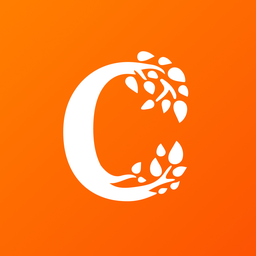
Full access? Get Clinical Tree
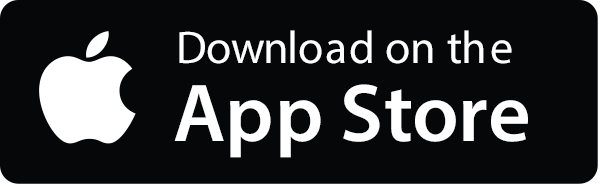
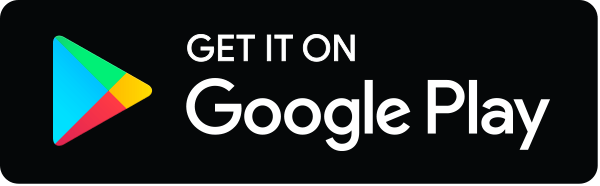