© Springer International Publishing AG 2017
Bernard Roger, Ali Guermazi and Abdalla Skaf (eds.)Muscle Injuries in Sport AthletesSports and Traumatology10.1007/978-3-319-43344-8_11. Muscle Physiology in Athletes
(1)
Service de Médecine du Sport, Centre Hospitalier de Perpignan, Perpignan, France
(2)
Clinic ProSport–Mon Stade, Paris, France
Abstract
Skeletal muscle exhibits plasticity in response to physical training. The functional consequences of these adaptations are determined by training volume, intensity and frequency. One could oppose the practice of endurance sports to sports involving strength and speed. Endurance exercise leads to physiological and biochemical adaptations in skeletal muscle which sustain aerobic metabolism capacity such as mitochondrial biogenesis, angiogenesis, and fiber type transformation. Strength training stimulates synthesis of contractile proteins that are responsible for muscle hypertrophy and increased maximal contractile force output. The increase in muscle mass observed in response to strength training is related to hypertrophy of cellular components, with an increase in their number referred to as hyperplasia. These adaptive changes are responsible for the improvement of physical performance. This review focuses on the mechanisms involved in these adaptations. Modifications of muscle typology under the effect of training result from three main factors: nerve stimulation, mechanical stress resulting from the type of physical activity, and the metabolic response to effort. Beside these main factors of muscle adaptation, hormonal response and nutrition can modulate their expression. Recent findings have revealed some of the mechanisms of various signal transduction pathways and gene expression programs in exercise-induced skeletal muscle adaptations. It is now possible to study the effects of various training interventions on a variety of signaling proteins and early-response genes in skeletal muscle. A practical question is whether it is possible to relate muscle structural and functional capacities to performance. Physiological and possibly pathological structural modifications are appreciated through the various imaging techniques, such as dual energy X-ray absorptiometry (DEXA), radiography and computed tomography, magnetic resonance imaging and ultrasound, all of which have been applied to the study of how changes in muscle mass are effected by training. Measuring cross-sectional surface area by means of ultrasound helps evaluate with precision the increase in segmental cross-sectional surface area. A non-invasive measurement of the effects of training on muscle typology can be realized using magnetic resonance spectrometry, 31P-MRS.
1.1 Introduction
To begin, a brief overview of the normal structural and functional mechanism of skeletal muscle is given.
1.1.1 Skeletal Muscle Function
The skeletal muscle is the only organ that ensures the biomechanical work of locomotion. It is able to transform the biochemical energy contained in energetic substrates into mechanical energy. Its structure and functional capabilities are adapted to the various types of constraints it endures. The level of physical activity, defined in terms of work load and intensity, influence the total muscle mass as well as the muscle’s metabolic and contractile properties. According to the type of physical training, muscle adaptations will be focused either on development of muscle power or the capacity to sustain prolonged work. This leads to the distinction between the effects of strength training and the effects of endurance training. Recently, our interest has centered on the effects of workouts that combine strength and endurance. The muscle’s ability to adapt to the various demands is referred to as muscle plasticity. To understand the hierarchy of such factors of muscle adaptation to training conditions, the first step is to give a short description of muscle structure and muscle contraction mechanisms. This serves as a basis for explaining the molecular mechanisms involved in muscle plasticity which are beginning to be well determined.
1.1.2 Muscle Fiber Composition
Myofibrils represent the histological entity of the striated muscle. The particular structure of the myofibrils gives the skeletal muscle its “striated” appearance. The proteins that make up such myofibrils are organized as sarcomeres delimited by Z-shaped striae. Sarcomeres are organized in series along the muscle fibers. From the sarcomere’s periphery to its center, there is a succession of I bands (clear, called isotopic) and A bands (dark, called anisotropic). I bands consist of fine filaments made up of two spiral chains of globular actin enabling control of the contractile property of actomyosin filaments by calcium. A bands consist of thick filaments of myosin molecules, the main contractile element of muscles.
1.1.3 Muscle Contraction Mechanism
Muscle contractions result from the transformation of chemical energy into mechanical energy through the slip of contractile protein filaments, actin and myosin. Chemical energy is provided by the hydrolysis of adenosine triphosphate (ATP) under the influence of the ATPasic activity of the myosin head. The hydrolyzing activity of this enzyme regulates the slip speed between the myofilaments. The contractile process mainly depends on the nerve command which regulates motricity at the central level of the organism. This nerve control is the contractility excitation factor which is based on the transmission of information from the muscle periphery to the inner area of the cell by coupling excitation and contraction. This coupling and the contraction-release cycle of the muscle fiber are directly related to an increase in the concentration of intracytoplasmic ionized calcium [32]. The rise in calcium produces an interaction between the actin molecules and the myosin head which conditions the slip of filaments and so ensures the mechanical phenomenon of muscle contraction. Release follows the contraction. After having been brought in contact with the ATPasic site of the myosin molecule head, the ATP is hydrolyzed and the link between actin and myosin is broken. This time corresponds to the re-uptake of calcium by the sarcoplasmic reticulum; these various stages are energy consuming. Power, resistance to fatigue, and contraction speed are dependent on the nature of contractile proteins and the metabolic resources of each muscle fiber. There are several types of muscle fibers that can be classified into different types based on their contractile and metabolic properties (reviewed by Schiaffino and Reggiani [41]). There are two major motor units: slow type motor units (slow type I), characterized by the slowness of their contraction, the low value of their mechanical power, and their resistance to fatigue. By contrast, fast type motor units are characterized by their fast contraction and high power. They are classified as fast-fatigable (fast twitch IIb/IIx) or fast-resistant (fast twitch IIa), according to their resistance to fatigue. IIx fibers are the fastest and have a strictly anaerobic metabolism. IIa fibers have a mixed aerobic and anaerobic metabolism. Contractile properties, notably the contraction speed of the motor unit, are closely dependent on the speed of ATP hydrolysis, which in turn, depends on polymorphism of contractile proteins. This variability is largely determined by the various types of myosin heavy chains. The type of fibers is determined through myosin heavy chains. The fibers listed as IIb in the classification based on ATPase coloring actually contain type IIx myosin heavy chains. In humans, only type IIx fibers are expressed. Each myosin molecule is formed by the association of two polypeptide chains said to be “heavy” that are called myosin heavy chains and four light polypeptide chains that are called myosin light chains. In humans there are three isoforms of myosin heavy chains–slow type I, fast types IIa and IIx. Slow type I is expressed in slow fibers and the two others in fast fibers. The various isoforms of light chains play a role in the sensitivity of the ATPase site of heavy chains by modulating sensitivity to calcium. Type I myosin heavy chain produces an actomyosin interaction which is slower than rapid type chains. The modifications of myosin isoforms are the most relevant indicators of muscle remodeling in response to training [43].
The metabolic properties depend on enzymatic resources and mitochondrial density. Type I fibers have a strong mitochondrial density and enzymes directing metabolism towards oxidative pathways. They are capable of using carbohydrate or lipid substrates and they are also the seat of the oxidation of certain amino acids during muscular work. Rapid fibers are classified into two sub-sets that differ in terms of their metabolic capabilities. Type IIa fatigue-resistant fibers are able to ensure a substantial oxidative metabolism, while type IIb/IIx fatigable rapid fibers have an essentially anaerobic metabolism.
1.1.4 The Capillary Network
The capillary network of the striated muscle is of paramount importance in as much as it represents the exchange interface between the vascular bed and the muscle fiber. It is the transport and distribution system of oxygen and substrates. The diffusion of capillary oxygen at the center of the fiber is an essential factor for muscle metabolism during the contraction phase and during recovery. It is essential to emphasize that the capillary layout is of considerable importance for the assimilation of substrates and the release of metabolites. The number of capillaries that come in contact with a fiber and thereby contribute to its supply depends on the metabolic property of the latter. Physical training increases capillary density in muscle fibers.
1.2 Effects of Physical Training on Muscle Structures
1.2.1 Effects of Endurance Training
When we attempt to map out the effects of training on muscles, we oppose the practice of endurance sports to sports involving strength and speed. The main adaptation of muscle to endurance sports results from the capacity to maintain a substantial flow of energy for a prolonged time without fatigue. Therefore, the use of ATP should be continually compensated by energy processes that balance energy supply and use. This process requires a sufficient oxygen flow and an availability of energy substrates in the muscle. Three major types of adaptations result from this type of training: an increase in mitochondria, a modification of muscle types, and orientation of the use of energy substrates. To fulfil such conditions, endurance training increases the density of mitochondria, spurs the activity of enzymes of the Krebs cycle, favors capillary proliferation around muscles, and increases intra-muscular glycogen and triglyceride reserves. The density of mitochondria increases relatively rapidly at the start of training. A 40 % increase in the total volume of mitochondria in the quadriceps of sedentary individuals can be seen after six weeks of endurance training [24]. This adaptation is partly responsible for the increase in maximal oxygen uptake. Increasing the volume of mitochondria per muscle unit allows preferential use of lipids which is facilitated by an increase in intra-muscle lipid content [26]. It has been well established that endurance training causes a transition from glycolytic type fast fibers (type II) to slower oxidative type fibers (type I). The effects of endurance training have been a controversial issue for many years. Early studies on the typical features of subjects according to physical activity showed that among the main locomotor muscles such as the quadriceps, endurance athletes had a predominance of type I fibers that could reach up to 90 % of total fibers whereas sprinters had a predominance of type II fibers. Such differences have been attributed to natural aptitude rather than training. Discussion remains open to what extent such muscle modifications in athletes who succeed in endurance disciplines is the result of training or genes [39]. This issue has been explored in studies of monozygotic and dizygotic twins [11], the results of which indicate that 25–50 % of the variability in muscle typology is hereditary. Among the many genes that have been identified as able to influence global response to training, a peroxisome proliferator activated receptor α (PPARα) can be isolated from the gene that regulates actinic production (ACTN3 gene); both genes are associated with muscle typology [37]. The α-Actinin-3 is a muscle protein that interacts with metabolic and nervous signals of adaptation to training. The ACTN3 577XX type polymorphism is more common in endurance athletes [16]. It has now been well established that endurance training on top of genetic factors causes a transformation of muscle fibers towards a predominance of slow fibers and also produces a transition from type IIb glycolytic fast fibers to type IIa oxidative fast fibers [42]. This type of transition is advantageous because it reduces muscular fatigue considerably. Oxidative slow fibers have a section surface weaker than fast fibers; this enables a better diffusion of oxygen towards the mitochondria but also has the disadvantage of reducing force capacity per motor unit.
1.2.2 Effects of Strength Training
Several factors determine strength production by muscles. These are successively the surface of the muscle section, the typology of the muscle fibers, the number of motor units solicited and an anatomic insertion parameter of muscles on the bone segments, known as the pennation angle. The ability of an athlete to develop significant strength mainly depends on structural and neural factors [9]. Structural factors relate to muscle composition itself whereas neural factors relate to the use of motor units. The increase in muscle mass observed in response to strength training is related to a hypertrophy of cellular components, with an increase in their number referred to as hyperplasia.
1.2.2.1 Hypertrophy
Muscular strength production depends directly on the muscle section [27]. Strength training rapidly produces an increase in the section surface of muscle fibers. Studies indicate an increase of 5–10 % in the lower limbs and 15–30 % on the upper limbs after 10–15 weeks of training [3]. Chronic muscle hypertrophy is an indication of structural modifications in muscles: multiplication and hypertrophy of myofibrils, and an increase in the connective tissue. This hypertrophy phenomenon is very important in athletes who have been undergoing strength training for several years and can be translated by an increase of over 80 % in the cross section surface of muscle fibers compared to the sedentary population. Hypertrophy relates to all types of muscle fibers with predominance of type IIb; the surface increase of IIa fibers is particularly clear in the upper limb muscles of weightlifters [28]. The hypertrophy mechanism results from the stimulation of satellite cells situated at the periphery of each fiber. Satellite cells are myogenic precursor cells situated between the sarcolemma and the basal plate of muscle fibers that induce the proliferation of the number of nuclei. The increase in fiber size results from the addition of the number of nuclei [51]. The same mechanism is involved in muscle repair after an injury [40]. This parallel action supports the theory that hypertrophy is regulated by the production of micro-lesions as a result of training and which, during repair, facilitate muscle hypertrophy [21]. Several studies underscore an increase in the contents of satellite cells in response to strength training [28]. However, this activation of satellite cells might have several physiological explanations. In one view, activation of such cells is an indication of their powers of regeneration in response to small lesions resulting from strength training. Or perhaps such activation is an indication of the incorporation of new nuclei by muscle fibers which could participate in addressing fiber synthesis needs and therefore muscle hypertrophy. Satellite cells multiply (proliferation step) and fuse with each other and with pre-existing muscle fibers (differentiation step) to enable muscle tissue regeneration [15]. The increase in muscle volume also results from the development of muscle envelopes as the connective tissue adapts to the mechanical constraints of training; the increase in the strength of this connective tissue is the consequence of the regeneration of muscle lesions due to physical exercise.
1.2.2.2 Possibility of an Increase in Muscle Cell Hyperplasia
The phenomenon of hypertrophy of muscle fibers is supported by multiplication of the muscle fibers: the hyperplasia phenomenon. The increase in the number of muscle cells in response to strength training was first demonstrated in animal models. In humans, the exact contribution of hypertrophy and hyperplasia in the increase of muscle volume remains controversial [19]. The mechanism responsible for this phenomenon could be stimulation of myogenesis by growth factors. Muscle hypertrophy and hyperplasia together make up a protective mechanism by which unusually intense contraction stimuli are distributed over a larger muscle mass, therefore offering relative protection against overload, as the load borne by each muscle fiber is reduced.
1.2.2.3 Effect of Strength Training on Muscle Typology
Muscle power is largely dependent on the maximum contractile speed. Apart from muscle mass itself, the muscle’s composition of the various types of fibers influences strength. The more important density in myofibrils of type IIA fast fibers and especially type IIX improve upon the speed and strength of muscle contraction. High level weightlifters who have regularly undergone strength training for several years have a higher percentage of type IIA rapid fibers and a lower percentage of type I slow fibers [28]. Change in density also leads to modification of muscular metabolism. Hypertrophy in response to strength training modifies muscular metabolism by reducing oxidative capabilities. These result from the decrease of mitochondria density in muscles, due to an increase in the quantity of myofibrillar proteins. The decrease in mitochondrial density is associated with a decrease in the activity of Krebs cycle oxidative enzymes [12].
1.2.2.4 Role of Nerve Stimulation
The observed rapid increase in strength at the beginning of strength training before the appearance of structural phenomena is attributed to nervous factors of adaptation to this type of training [20]. Such neural factors are highlighted by the increase in EMG activity which indicates an increase in the number of recruited motor units and an improvement of motor unit synchronization [35]. Motor units are recruited according to their size, from smaller to bigger, referred to as Henneman’s size principle [22]. Subjects who undergo strength training are therefore able to activate a more important number of motor units within the solicited muscle, thus allowing them to develop greater strength. The structures responsible for this adaptation are situated at the peripheral stage of the neuromuscular junction but also at the cortical level of the motor command [17]. This adaptation occurs on a very short term after only a few weeks of training and is associated with an increase in the stiffness of the muscle-tendon torque, which favors muscle performance [50].
1.2.2.5 The Role of Muscle Architecture
Muscle contraction strength is influenced by a stress-length relation. For each muscle fiber there is an optimal tension which, according to the initial model by Sonnenblick and Skelton [46], enables a greater juncture between actin and myosin filaments. Anatomically, the arrangement of individual fibers affects their traction angle (pennation) as well as the articulation angle which influence muscle length and the capacity to produce strength and contraction speed. The decrease in pennation angle helps obtain an optimum length when relaxed to favor contraction speed. The longer the fibers are, the more they have of sarcomeres in series, which is favorable to speed; sprinters have more lengthening of muscle fibers than long distance runners [2]. Kumagai et al. [33] showed significant differences in the pennation angle between two groups of sprinters: 10 s sprinters have longer fiber bundles than 11 s sprinters, as well as a lower pennation angle and are more effective at producing speed. Moreover, the pennation angle also increases total muscle mass connected to a tendon [29]. This has a genetic aspect but may also be a result of training. Muscle hypertrophy in response to strength training is associated with a decrease in the pennation angle [1].
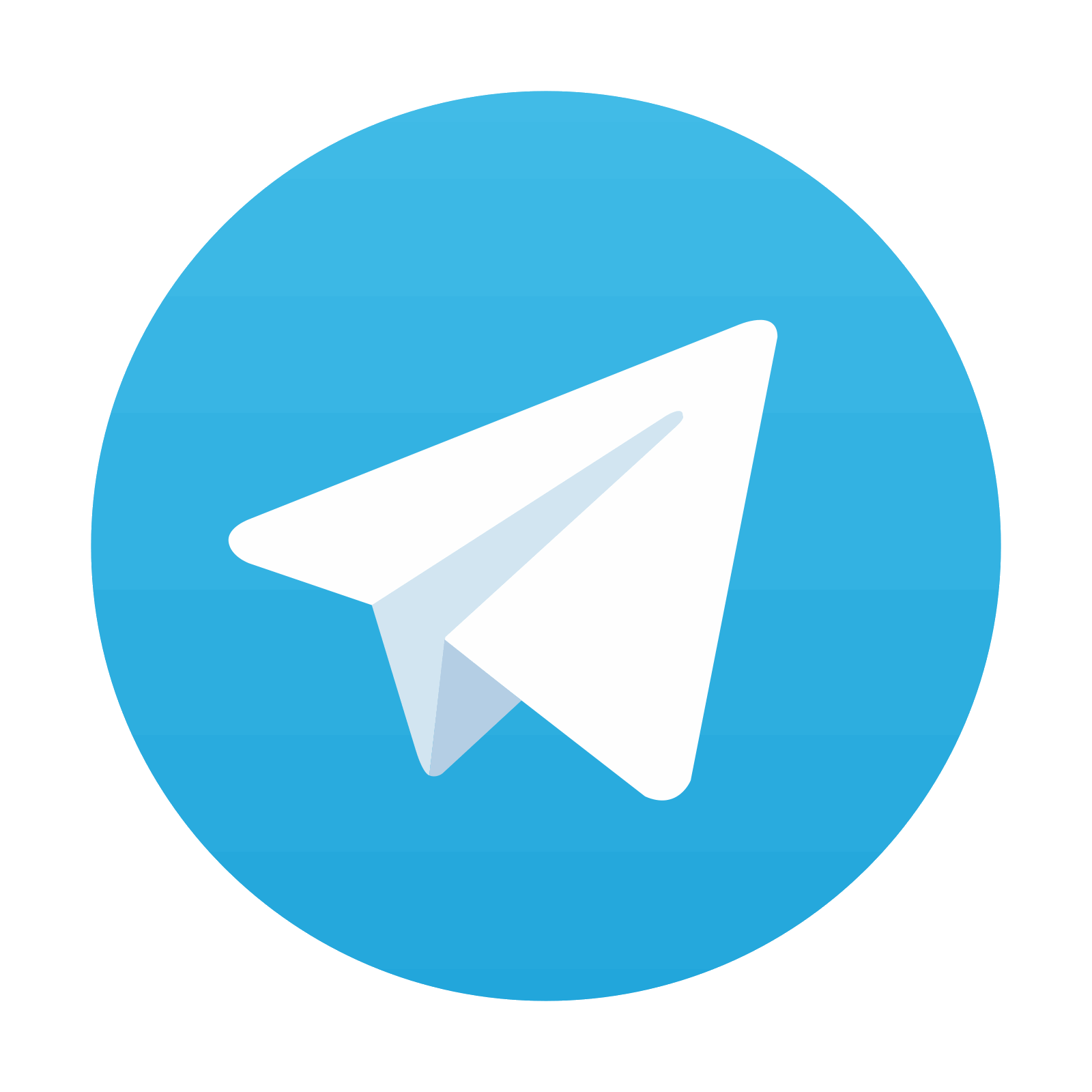
Stay updated, free articles. Join our Telegram channel
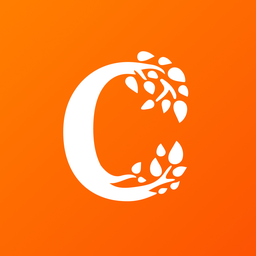
Full access? Get Clinical Tree
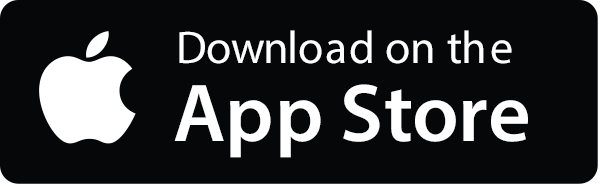
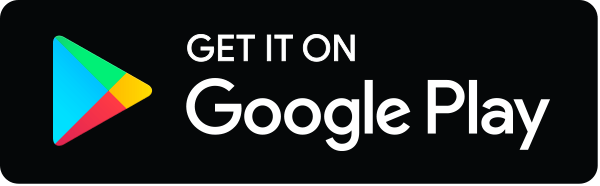
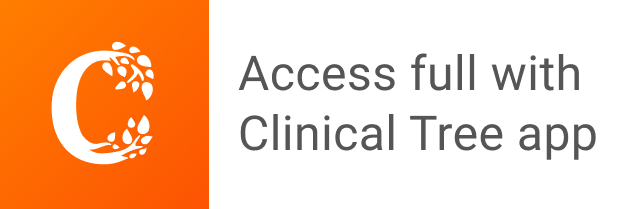