Chapter 5 Muscle Fatigue
After studying this chapter, the reader will be able to do the following:
A strict definition of muscle fatigue has been difficult to establish but may be defined as the inability of muscle to maintain the expected force or power output, resulting in a decline of maximum force-generating capacity.1–4 Although it may be easy to loosely define fatigue, the question “What causes muscle fatigue?” is infinitely more difficult to answer. Due to the complexity, intensity, and repetitive nature of activities and tasks in general, many components can potentially influence both the onset and progress of fatigue. For example, fatigue may depend on a person’s state of fitness, diet, sex, age, health status, overall body composition, fiber type composition, muscle groups involved, the type of sport being performed, the specific task at hand, or the physical and chemical composition of the environment in which the task is performed.5,6 Fatigue during voluntary muscle contractions can be a complex and multifaceted phenomenon, and researchers are just beginning to understand some of the specific mechanisms involved. Consequently, identification of a single definitive mechanism may be somewhat unrealistic.7 In addition, motivation can be a crucial factor, allowing the individual to push beyond the discomforts of fatiguing exercise. Motivational factors are undoubtedly more difficult to categorize or quantify but play a major role when attempting to achieve superior performance. An in-depth discussion of motivational factors is not discussed here.
EXAMINATION OF MUSCLE FATIGUE MECHANISMS
In vitro investigations attempt to study function by simulating various intracellular environments using test tubes. This allows researchers to address mechanisms, such as metabolite accumulation, activation failure, substrate depletion, impairment of calcium (Ca2+) kinetics, and disruption of the myofibrillar complex.8 For example, when studying muscle fatigue, researchers may use fractionated muscle tissue to examine the function of specific organelles, such as the sarcolemma, the sarcoplasmic reticulum (SR), or the myofibrillar complex (i.e., actin-myosin cross-bridge dynamics) and their role in energy metabolism. With this approach, fatigued muscle is compared with fresh muscle in order to determine differences between the two as a result of exercise.9 Of course, caution must be observed when interpreting the results because it is quite possible that the organelles may be disrupted by the preparation or possibly only partially recovered.
Additionally, researchers may study the muscle tissue itself, without disruption, using in vitro techniques. In this case, either the whole muscle, a bundle of muscle, or single muscle fibers dissected from an experimental animal are placed in a physiological medium and connected to a force transducer.8,9 Moreover, single fibers may be examined with an intact sarcolemma or with the sarcolemma removed. Examining the fiber with the sarcolemma removed, known as the skinned fiber technique, allows the researcher to directly manipulate the intracellular environment.9 In other words, the researcher can put holes in the membrane and directly add ions to the fiber to observe force changes. Although these experiments do help researchers understand muscle fiber physiology, they may not necessarily identify those rate-limiting mechanisms that occur with human performance.
In vivo experiments, using whole animal preparations, may be advantageous because these experiments use intact tissue and presumably do not disturb the intracellular organization of the fiber. Induced or imposed contractile patterns are used with these preparations, resulting in an earlier and more pronounced fatigue than when using voluntary activity at the same mechanical demands.9,10 In these preparations, the stabilized, anesthetized limb is isolated and prepared for mechanical simulation using different protocols. Measurement of fatigue manifestations can then be characterized. Because experiments may take several hours, the main concern with these studies is the physiological viability of the preparations while waiting for the experimental protocol to take place.
Fatigue studies can also be performed using single motor units, in which the motor units can be isolated using dorsal root dissection; this may allow the researcher to relate specific alterations in motor unit function with changes at the level of the single fiber.9
MECHANICAL MANIFESTATIONS OF MUSCLE FATIGUE
Before changes in specific intracellular processes can be determined to contribute to fatigue, the mechanical parameters must first be defined. When discussing fatigue, identifying the underlying mechanical basis is crucial. Determining what type of exercise or task is performed is important because fatigue may develop secondary to failure at one particular site or at a combination of sites along the pathway of force production.1
A common complicating factor when studying human fatigue concerns the motor unit activation pattern. Motor unit recruitment simply means adding motor units to increase muscle force. For example, low-force muscle activity requires only a few motor units to be activated, whereas a higher-force activity requires progressively more motor unit enlistment. Additionally, as motor force increases, motor neurons with progressively larger axons are recruited.11 Most muscles are composed of a mixture of different fiber types. However, all motor units in a muscle do not fire at the same time; this allows a smooth control of muscle force output.
Evidence-Based Clinical Application: Motor Unit Recruitment
Because muscles are composed of motor units and each motor unit is essentially recruited in an orderly fashion (depending on the task), fatigability may be enhanced in some motor units more than others with all fiber types being recruited during intense activity.12,13
The recruitment sequence reflects the order of threshold for activation. In this case the slow-twitch Type I fiber (lowest threshold for activation) is more fatigue resistant than Type IIA, which is more fatigue resistant than Type IIX (intermediate fiber), which is more fatigue resistant than Type IIB (fast-twitch fatigable).14,15 Performance of submaximal contractions usually involves recruitment of Type I (and possibly Type IIA) fibers from the onset of the activity. Exercise progression results in an increasing number of Type II fibers being recruited until exhaustion, when all motor units have been brought into play.
In using the simple model of isometric activity, if the contraction is intense, a tetanus or fused contraction is produced, allowing the site of fatigue to be easily identified because only one mechanical parameter is involved.9 However, at lower intensities of contraction (oscillatory nonfused contractions), other factors that might impair performance, such as contraction time and relaxation time, become involved.
UNDERLYING CAUSES AND SITES OF MUSCLE FATIGUE
Muscle fatigue can be broken down essentially into two main components: central (neural) and peripheral (muscular). Peripheral fatigue refers to the motor units and involves processes associated with mechanical and cellular changes in the muscular system.11 Central fatigue refers to the physiological processes that occur within the central nervous system (CNS). Although many researchers would agree that both peripheral and central factors play a role in muscle fatigue, the relative contribution of both may vary and remains poorly understood. Following is a brief discussion of the various aspects of neuromuscular fatigue and the mechanisms involved.
PERIPHERAL FATIGUE
When examining peripheral fatigue, the goal is to specify what process in excitation and contraction of the muscle represents the primary site(s) of failure. As such, peripheral fatigue may involve the following sites and pathways: (1) neuromuscular junction, (2) the excitation-contraction (E-C) coupling process, and (3) the activation of the contractile elements involved in force and power generation.14 Contractile force can decrease with interruption of any one of these processes.
With voluntary activity, processes involved in force production can be located outside the muscle (central processes) or in the muscle. Because the nerve ending terminates at the neuromuscular junction and further invaginates into the muscle fiber, the neuromuscular junction is considered for this discussion as part of the peripheral mechanism, although it may be argued that it is under the control of central processes. These outside processes may include events leading to muscle fiber recruitment involving the alpha motoneuron, potentially altering its excitability. In this case motor nerve impulses may be distorted because of branch point fatigue or inhibition at the neuromuscular junction.16 In other words, fatigue can occur at the neuromuscular junction if an action potential (AP) fails to adequately reach the muscle fiber from the motor neuron.
Fatigue may also come about with nerve fiber stimulation at rates greater than 100 times per second. In this case there is a decreased number of acetylcholine vesicles released with each impulse that ultimately fails to pass into the muscle fiber, leading to fatigue of the neuromuscular junction.17 Of course, this would probably only occur at extreme levels of muscular exhaustion.
The E-C coupling process can be broadly defined as the complex signaling process from nerve depolarization to muscle contraction.18 Basically, excitation sites include the sarcolemma and the transverse tubule (t tubule) system, which are specialized to conduct the electrical signals into the muscle fiber’s interior. In order for a contraction to take place, the signal must result in the opening of the Ca2+ channels in the SR, leading to a release of the stored Ca2+ into the cytosol. But to do this, the signal in the t tubule membrane must communicate with the Ca2+ channels in the terminal cisternae of the SR (traversing an approximate 10 to 15 nm gap).9
The E-C coupling process begins with transmission of APs down the motor neuron axon, thereby activating the surface membrane. Then the alpha motoneuron releases acetylcholine at the neuromuscular junction, resulting in binding of acetylcholine to its receptor and depolarization at the end-plate, which opens the voltage-gated Na+ channels. This leads to depolarization of the sarcolemma, resulting in propagation down the t-tubules (within the sarcolemma) and subsequent release of Ca2+ from the SR (the terminal cisternae) into the cytosol. Here Ca2+ can bind to troponin C (on the thin filament) and initiate cross-bridge cycling.19 Consequently, muscle relaxation occurs by reuptake of Ca2+ into the SR and subsequent deactivation of the actin-myosin cross-bridges. Disruptions in E-C coupling would involve events affecting the level of Ca2+ in the cytosol. Alterations in either the release of Ca2+into the cytosol or its subsequent sequestration back into the SR may affect the myofibrillar complex, resulting in fatigue.
Another potential fatigue site involves the myofibrillar complex. This complex, representing the contractile apparatus, includes the regulatory proteins troponin and tropomyosin, as well as the contractile proteins actin and myosin and their cross-bridge interaction. Revisiting the E-C coupling process, if the amount of Ca2+ released from the SR into the cytosol is diminished, the binding of Ca2+ to troponin C will be attenuated. The inability of the regulatory proteins to respond to the Ca2+ signal or the inability of the actin and myosin to transition to different binding states could cause a decrease in force to occur. Consequently, fewer cross-bridges will be formed, resulting in a lower force or power generation.15 Additionally, cross-bridge cycling depends on an adequate supply of adenosine triphosphate (ATP) through aerobic and anaerobic metabolic pathways.
The Sarcoplasmic Reticulum, the Sarcolemma-T-Tubule System, and Muscle Fatigue
The sarcolemma and the t-tubules both contain resting membrane potentials and are capable of being depolarized and conducting an AP. In essence, they represent an electrical pathway whereby motor nerve excitation via the neuromuscular junction and motor end plate is transported to specific intracellular sites.9 Thus when an AP occurs, it spreads over the sarcolemma and courses deep into the interior of the muscle fiber via the t-tubules causing a change in the permeability of the TC and permitting Ca2+ to escape, thereby eliciting a muscle contraction.20 As such, the force developed by a muscle fiber is closely dependent on the frequency with which APs can be regenerated in the sarcolemma and t-tubules.
The generation of an AP depends on the opening of the Na+ and K+ channels (Na+ to the interior of the cell and K+ to the interstitial space) and the ability to quickly reestablish the Na+ and K+ gradients along the membrane. The reestablishment of these ionic gradients is controlled primarily by the ATPase pump, which is triggered by the Na+ and K+ concentrations on the interior and exterior of the cell, respectively, resulting in increased cycling.9 This process depends on the use of energy generated by the hydrolysis of ATP.21,22 However, it should be noted that a suppression in ATPase activity could be a result of increased by-products of ATP hydrolysis (i.e., phosphate and hydrogen ions). These ions would alter the ATPase activity once certain levels were attained.23 If the sarcolemma and t-tubules are to conduct APs at a rate necessary to maximally activate the fiber, then the ATPase pump must have high ATPase activity and an increased capacity for rapid ATP hydrolysis.7
An inability to repeatedly generate APs at high frequency for maximal force generation by the fiber may result in excitation failure, referred to as high-frequency fatigue.24 This fatigue seems to occur due to an inability to restore NA+ and K+ gradients across the sarcolemma before the next AP. An inability to reestablish these gradients could alter the membrane potential and compromise force production secondary to potentially impairing the ability of the t-tubule to signal the SR.
Three key issues are of importance involving the SR, which may possibly determine its vulnerability to fatigue. The first issue concerns the storage capability of the SR for Ca2+, which is sequestered when the muscle is quiet. Secondly, the SR must be able to release the stored Ca2+ into the cytoplasm on excitation, which occurs primarily at the TC (the Ca2+ storage site). Thirdly, the SR must be able to sequester the Ca2+ via the transporting enzyme Ca2+-ATPase back into the SR against an electrochemical gradient, which requires energy. Sequestration is necessary for relaxation of the muscle fiber following excitation.9
Skeletal muscle displays wide variability in mechanical function. Research has shown differences in the ability of fast- and slow-twitch muscles to activate the Na+-K+ pump.25 Consequently, the speed which with a fiber responds to different excitation patterns varies. For example, fast-twitch fibers contract and relax much faster than slow-twitch fibers because the SR in fast-twitch fibers is more extensive and they have a higher density of both Ca2+ channels and Ca2+-ATPase enzymes. This may help to explain some of the differences noted in fatigability.9
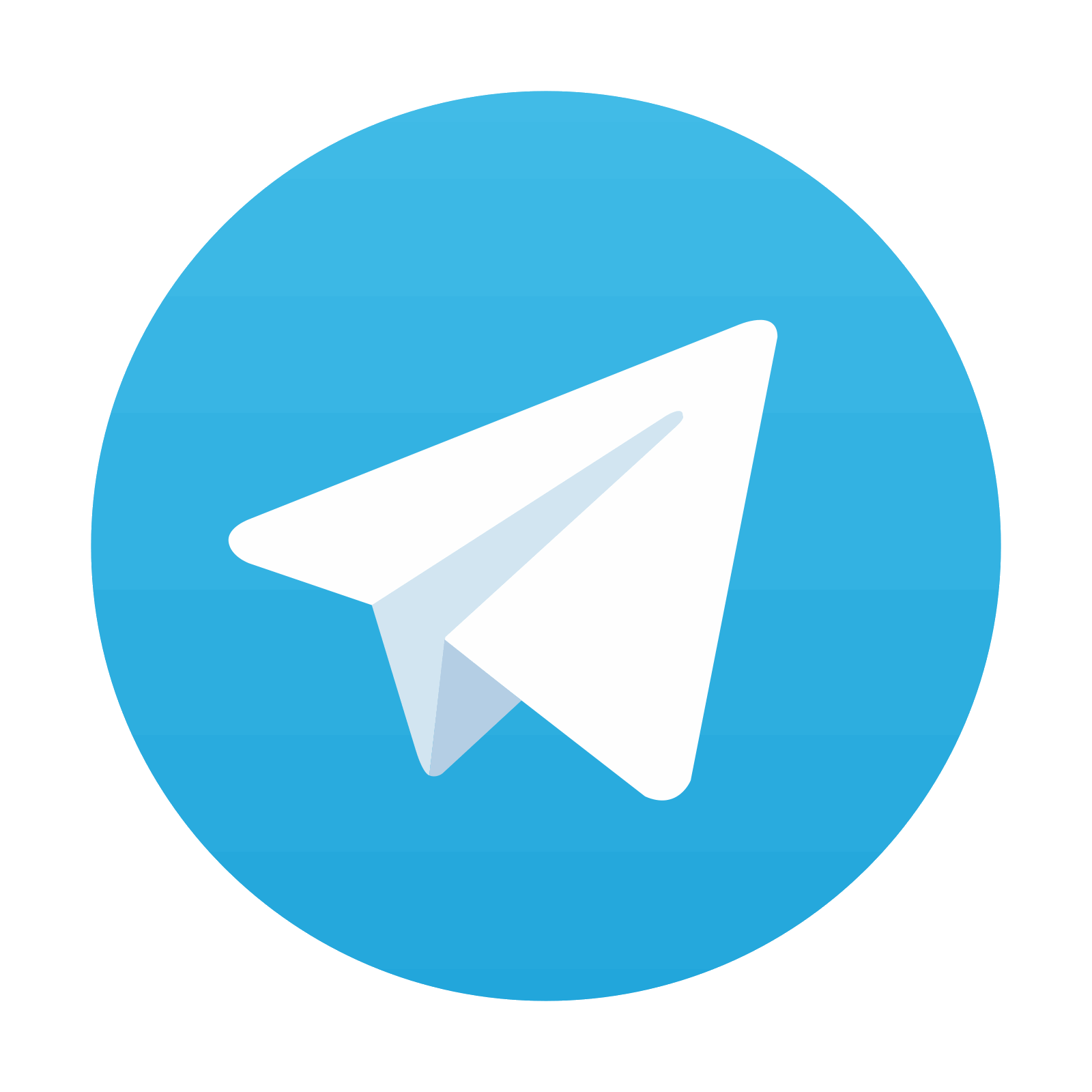
Stay updated, free articles. Join our Telegram channel
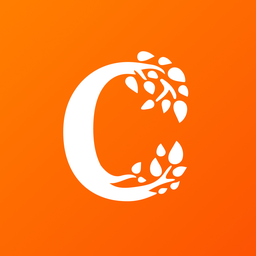
Full access? Get Clinical Tree
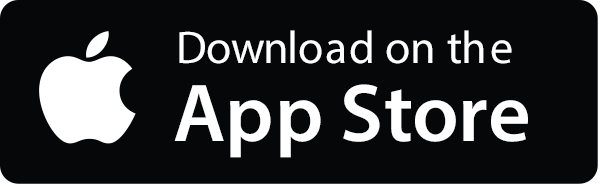
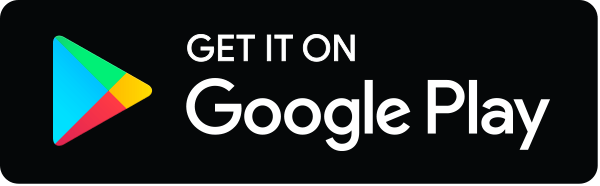