© ISAKOS 2017
Alberto Gobbi, João Espregueira-Mendes, John G. Lane and Mustafa Karahan (eds.)Bio-orthopaedics10.1007/978-3-662-54181-4_77. Host Environment: Scaffolds and Signaling (Tissue Engineering) Articular Cartilage Regeneration: Cells, Scaffolds, and Growth Factors
(1)
RAMSES Laboratory, Rizzoli RIT—Research, Innovation and Technology Department, Istituto di Ricerca Codivilla Putti, Istituto Ortopedico Rizzoli, Via di Barbiano, 1/10, 40136 Bologna, Italy
(2)
Laboratory of Immunorheumatology and Tissue Regeneration, Istituto di Ricerca Codivilla Putti, Istituto Ortopedico Rizzoli, Via di Barbiano, 1/10, 40136 Bologna, Italy
7.1 Introduction
7.1.1 Articular Cartilage Biology
Articular cartilage covers the ends of bones in diarthrodial joints. Histologically, it is a hyaline tissue with no blood, lymphatic or nerve supply [1].
Hyaline articular cartilage is a highly specialized tissue derived from mesenchyme during embryonic development. It has the main function to protect the joint by distributing loads and thus preventing potentially damaging stress on the subchondral bone. At the same time, it provides a low-friction bearing surface to enable free movements. The tissue is composed of two phases: liquid and solid. Generally, 60–80% of total wet weight of articular cartilage is fluid (e.g., interstitial water and electrolytes). The remaining 40–20% of the tissue is composed by a solid extra cellular matrix (ECM) and chondrocytes [2].
Chondrocytes, the only cell type of mature cartilage, secrete, and deposit around themselves a characteristic ECM. Moreover, they maintain and remodel the ECM by a careful balance of catabolic and anabolic processes, involving matrix metalloproteinases (MMPs) and tissue inhibitors of metalloproteinases (TIMPs) [3]. Mature chondrocytes are completely encapsulated in the dense cartilage ECM and are not able to migrate or proliferate, unlike cells in bone.
The ECM is composed primarily of water, collagens, proteoglycans, and other non-collagenous proteins. Besides collagen type a variety of collagens such as types VI, IX, X, and XI are present and contribute to the tensile properties of articular cartilage. In adult tissue, collagen type II represents 90% of total collagens. Conversely, the proportion of collagen XI is about 3%. All collagen fibers display oriented distribution and nonuniform size. Proteoglycans are mainly represented by aggrecan that provides compressive properties to the tissue. Aggrecans exhibit a bottlebrush structure, in which glycosaminoglycan (GAG) chains (chondroitin sulfate and keratan sulfate) are attached to a high molecular weight protein core. Such a structure aggregates with hyaluronic acid, known also as hyaluronan (HA), via a link protein. Aside from aggrecan, smaller proteoglycans like biglycan, fibromodulin, and decorin also occur in minute amounts [2]. ECM can be classified into three regions: pericellular, interterritorial, and territorial, based on their distance from chondrocytes. The thin pericellular matrix closely surrounds individual or a column of chondrocytes; the interterritorial matrix is farther, the territorial matrix is the farthest.
Mature articular cartilage, despite its thinness, presents a non-homogeneous distribution of cells and ECM among different zones, termed the superficial, middle (or transitional), deep (or radial), and calcified zones. In particular, when examined from the superficial to the deep zone: collagen and water contents gradually decrease; collagen fiber size increases; proteoglycans augment, with the middle zone having highest content. The superficial zone contains fibroblast-like chondrocytes that are flattened and oriented in parallel with the surface. Collagen fibers display a tangential orientation. In the middle zone, chondrocytes exhibit a round morphology; collagen fibrils have a random orientation. The deep zone displays chondrocytes packed in columns which remain parallel to the collagen fibers organized in radial directions. The calcified zone contains few inert chondrocytes embedded in a calcified ECM, having collagen type X, which helps cartilage mineralization and provides structure integrity.
Articular cartilage possesses significant mechanical properties with a compressive modulus of 0.7–0.8 MPa, a shear modulus of similar magnitude (0.69 MPa), and a tensile modulus of 0.3–10 MPa. Such mechanical properties are reminiscent of a hydrogel [4].
Despite its durability and ability to maintain itself, articular cartilage’s is vulnerable to injuries that lead to irreparable tissue damage, causing, over time, inflammation, disability, and pain. This originates from articular cartilage’s weak capacity for self-repair, which is due to its avascularity. Wound healing is further prevented due to the dense ECM, impairing chondrocyte migration capacity.
7.1.2 Defects of Articular Cartilage
Articular cartilage lesions are areas of damaged or missing tissues that can be of traumatic or rheumatic origins [5].
Traumatic alterations are common in the general population and more often anticipated in young and physically active people. They can be divided into microtraumas, chondral, and osteochondral defects. Microtrauma or light repeated trauma could provide degeneration or cell death, collagen ultrastructure destruction, hydration increase, and superficial subchondral bone fissuration. These phenomena are similar to those of the early phase of osteoarthritis (OA). Chondrocyte ability to synthesize the ECM is not sufficient to counteract the progress of the degenerative process that results irreversible. Common chondral defects enclose chondromalacia/degenerative chondrosis, chondral flap, and chondral fracture. In chondromalacia, cartilage tears away unevenly, with shallow walls; in chondral flap, the tissue separates from the bone and moves like a door with a hinge at one end and in chondral fracture cartilage is removed from the bone and floats free. Chondral lesions may be degenerative, due to a “wear and tear” problem or traumatic, caused by an injury such as falling on the knee, jumping down, or rapidly changing direction while playing a sport. Such problems do not always produce symptoms at first because there are no nerves in the cartilage. Moreover, in general, no repair process occurs. Osteochondral defects involve both the articular surface and the subchondral bone. The degree of injury ranges from a small crack to a piece of bone breaking off inside the joint. These fragments, differing for size and depth, can stay attached (stable) to the area that was injured or become loose (unstable) within the joint. Those kind of injuries are more common in adolescents and young adults and typically occurs at the knee, ankle, or elbow. In osteochondral defects, a repair process is initiated by undifferentiated mesenchymal stem cells (MSCs) from the bone marrow tissue of the subchondral bone. Repair of full-thickness cartilage lesions depends mainly on the patient age, defect size, and location. Small full-thickness defects are repaired by the formation of hyaline cartilage, whereas large osteochondral defects are only repaired by the formation of a scar, fibrous tissue, or fibrocartilage in which the predominant component is collagen type I. From a clinical point of view, this kind of cartilage could be functionally active for a short period, but the tissue does not present the mechanical and strength characteristics of normal cartilage thus ensuring neither the healing of the defect nor the symptom remission. This situation could favor over time the appearance of OA [6].
Articular cartilage is prone to rheumatic pathologies, such as OA and rheumatoid arthritis (RA) that compromise not only the structural and functional characteristics of the tissue, but also of the whole joint [5].
OA is a group of progressive joint disorders involving articular cartilage, subchondral bone, ligaments, and synovial membrane and resulting in patient disability. Both systemic and local factors could contribute to its development. Systemic factors include: age, sex, racial, and genetic characteristics and still unidentified factors. Local factors comprise articular traumatic injuries and deformities (dysplasia), obesity, and muscle weakness. OA highest prevalence before 50 years is dependent from sex, while after 50 years females are more affected. Advanced stages of the pathology lead to the need of prosthesis surgical interventions that, in these cases, represent the only therapeutic approach to restore the articular functions [5].
RA is an autoimmune, chronic, systemic, and inflammatory disorder. It principally attacks synovial joints, but it may affect also many other tissues and organs. Such a disease is associated with progressive joint destruction that leads to pain and disability. It can affect people of all ages and races and is more common in women. The cause of rheumatoid arthritis is not known, but in some families, multiple members can be affected, suggesting a genetic basis for the disorder [7].
7.1.3 Management of Cartilage Lesions
Despite being a known problem for a long time, cartilage restoration is a relatively new field in orthopedics. When developing such an approach, multiple factors should be considered: patient profile, age, type of lesion, concomitant factors, activity level, and expectations. The situation is further complicated by the lack of ability to self-repair of the tissue. For these reasons, there is no uniform approach to managing cartilage defects and a variety of surgical options have been developed [8]. These procedures can be classified as palliative, reparative, restorative, and regenerative, these last including the tissue-engineering-based strategies.
Palliative strategies are usually the primary approach to treat symptoms, but they do not lead to cartilage healing. Conservative approaches, such as physiotherapy, weight loss, intra-articular injections (analgesics, and viscosupplementation with glucosamine and chondroitin sulfate), and orthotic interventions are widely diffused. Debridement is utilized to remove all the debris deriving from the arthritis-mediated joint damage: inflammatory cells, unstable chondral flaps, osteophytes, superfluous synovia, degenerated menisci, and torn ligaments. Chondral shaving consists in the excision of damaged cartilage due to trauma. Knee joint lavage, a simply rinsing of the joint with physiological fluids to wash out degradation products, is usually performed when conservative treatment of knee OA is inadequate and replacement is not yet indicated [9].
Reparative approaches, known as abrasion, drilling, and microfracture, are aimed at the reconstruction of a new tissue that fills the defect site. In particular, they all enclose a phase consisting in the subchondral bone penetration, thus inducing bleeding. For this reason, these surgical strategies are commonly referred to as “marrow stimulation techniques.” As a consequence of drilling, there is a migration of bone marrow stem cells to the site of injury, along with blood clot formation. The resulting repair tissue is mainly composed of fibrocartilage. Although excellent short-term clinical outcomes have been demonstrated [10], the clinical durability of marrow stimulated repair tissue has shown a functional decline with further follow-up.
Restorative techniques’ purpose is the reconstitution of cartilage structure and function. Knee arthroplasty is widely diffused technique that is currently recommended for elderly patients. It has been shown to relieve pain and improve mobility in people suffering from end-stage lesions. However, it is an invasive procedure involving articular surface replacement with prosthetic implants. Complications may be stiffness, instability, aseptic loosening, infection, prosthesis failure, and malalignment [8].
Biological, regenerative approaches include osteochondral grafting and autologous chondrocyte transplantation (ACT), aimed at the formation of a new tissue that is indistinguishable from native cartilage. Osteochondral transplantation can be autologous (mosaicplasty) or allogeneic (allograft transplantation). Main mosaicplasty drawbacks are donor-site morbidity and graft failure due to marginal chondrocyte death. Limitations of allograft transplantation include graft availability, possible disease transmission, and a short cell viability and biomechanical integrity of the donor graft. For this last reason, fresh osteochondral allografts are recommended [11–14].
ACT was first used by a Swedish Group [15] to treat full-thickness chondral defects of the knee. In the original procedure (first-generation technique), small grafts of normal cartilage removed from non-weight bearing areas of the knee were treated in a proper laboratory. Individual chondrocytes were isolated, cultured in specific conditions, and, following a period of cellular division, retrieved for re-implantation. Cell suspension was then injected beneath a periosteal patch harvested from the proximal medial tibia and sutured to the defect. First-generation ACT revealed, however, several disadvantages [6]. Therefore, variations of the technique and new strategies have been developed, converging in the tissue engineering approach, which associates to the cells biomimetic scaffolds that can also be treated with soluble or mechanical stimuli. Moreover, researches have also been addresses towards the evaluation and development of cell sources alternative to chondrocytes.
7.2 Tissue Engineering and Cartilage Regeneration
The field of tissue engineering is highly multidisciplinary and draws on experts from clinical medicine, biological and materials sciences, and mechanical engineering.
Regenerative medicine deals with the process of regenerating cells, tissues, or organs to restore or establish normal function. In this perspective, tissue engineering relies on the use of porous three-dimensional (3D) scaffolds to provide the appropriate environment for the regeneration of tissues and organs.
The scaffolds, essentially acting as a template for tissue regeneration, can be utilized alone (acellular system) or in combination with cells and/or growth factors (GFs) or biophysical stimuli (i.e., bioreactor). The engineered cell-seeded scaffolds can be either directly placed into the injured site or pre-cultured in vitro before implantation.
7.2.1 Scaffolds
Scaffolds play a critical role in providing a 3D environment to support cell growth, matrix deposition, and tissue regeneration. An ideal scaffold should satisfy several essential criteria that concern its biological, structural, composition, and fabrication properties that make it available for tissue engineering applications:
Be biocompatible
Be biodegradable, with a favorable resorption rate
Not elicit an immune response
Be biomimetic, i.e., to reflect the 3D network structure of native cartilage
Display a porous structure (controlled pore size, distribution, and interconnectivity) to allow cell colonization and exchange of nutrients and wastes
Possess mechanical properties to support tissue growth under native mechanical loads
Promote cell viability, differentiation, and ECM production
Adhere and integrate within the host environment
Fit the defect size
Be easy to handle in the operating theater
Be simple to prepare
Be easily available
Be cost-effective
Currently, scaffolds suitable for cartilage tissue engineering can be divided into native biological and synthetic polymeric materials. Each type of scaffold presents specific advantages and drawbacks. Therefore, future research priorities need both to improve existing materials and fabrication techniques and to further develop new ones. In particular, recent developments in the manufacturing techniques allowed the creation of new types of scaffolds, such as nanostructured materials that are now in the spotlight for their potentiality in cartilage tissue engineering [2, 3, 16].
7.2.1.1 Natural Origin Scaffolds
Natural biomaterials are the most popular scaffolds for cartilage repair and regeneration due to their good biocompatibility. They include scaffolds based on HA, collagen, agarose, alginate, and chitosan [9].
HA has been extensively utilized to support chondrocyte growth or stimulate MSCs towards chondrogenesis because of its multiple functions in regulating and stabilizing the internal environment of cartilage. HA is an anionic, non-sulfated GAG whose properties in enhancing cartilage formation and regeneration are well documented. In particular, it was demonstrated that it protects chondrocytes from death receptor-induced apoptosis via the CD44 receptor. As a consequence, anabolic factors are activated, inducing cell differentiation [17]. However, natural HA is quickly degraded and resynthesized by the body; therefore, stabilization, by means of structure modification, is required to obtain a scaffold suitable for tissue engineering applications. Many different forms, preparations, and modifications exist, such as hydrogels, sponges, and meshes.
Collagens, the main protein components in natural cartilage, display excellent tissue compatibility and biodegradation, without provoking inflammation. It has been demonstrated that it is able to favor the adhesion of several cell types and to provide a stable environment to induce chondrogenesis or preserve the chondrocyte phenotype, both in vitro and in vivo. Gelatins, produced through the partial hydrolysis of collagen, display a similar chemical composition, but lack its antigenicity and immunogenicity. For this reason, they are largely utilized in tissue engineering applications [3, 18].
Agarose and alginate, both polysaccharides derived from seaweed, have been used to encapsulate cells for cartilage tissue engineering. However, despite the excellent cytocompatibility, they showed poor degradation properties [2].
Chitosan, a deacetylated derivative of chitin, has been extensively used in cartilage tissue engineering applications, alone or in combination with other materials, to enhance its properties. For instance, the hybridization of chitosan with HA displays several advantages, in comparison with chitosan alone. In particular, it improves chondrocyte adhesion to the scaffold, increases the proliferation rate while maintaining the round cell morphology, and enhances the accumulation of aggrecan and collagen II [2].
7.2.1.2 Synthetic Origin Scaffolds
The most popular synthetic polymers utilized for cartilage tissue engineering scaffolds are poly-lactic acid (PLA, both l and d forms), poly-glycolic acid (PGA), poly-caprolactone (PCL) and their copolymers such as poly-lactic-co-glycolic acid (PLGA). They are all characterized by the ease of fabrication, good biocompatibility, biodegradability, and suitable mechanical properties. Moreover, they can display porosity and surface structure suitable for cell attachment, proliferation, and differentiation. However, a critical drawback is the lower ability to interact with the cells, in comparison with natural polymers, that, owing to their intrinsic nature, show better bioactive properties. Therefore, composites combining respective advantages have been developed. For instance, fibrin glue, alginate, and HA have been used to modify various PLGA, PGA, and PCL scaffolds, and the results revealed that these composites can stimulate chondrogenesis [2].
7.2.1.3 Nanostructured Scaffolds
Nanostructured scaffolds have recently emerged as promising biomimetic candidates since cartilage itself is a typical example of a nanomaterial. In general, tissue-engineered scaffolds are able to recreate the macro- and micro-scale properties of the native tissue, while the nanoscale dimension, known to be crucial for cell function regulation and ECM synthesis, has been mostly neglected. The commonly accepted definition of nanomaterials refers to materials with clearly defined features between 1 and 100 nm, such as nanopatterns, nanofibers, nanotubes, nanopores, nanospheres, and nanocomposites. An example is a nanofibrous PCL scaffold composed by nanofibers and produced by the electrospinning technique that demonstrated to effectively induced MSC chondrogenic differentiation. In addition, the nanofibers displayed good mechanical properties [19].
7.2.1.4 Bioprinted Scaffolds
An interesting advancement that is rapidly evolving also in cartilage tissue engineering field is the bioprinting technology. This can be defined as a fabrication technique implying the layer-by-layer deposition of a mixture of cells and biomaterials. The procedure is innovative for several reasons. First of all, it allows the production of a structure by depositing together, in one single step, biomaterials and cells, while the conventional approach consisted in the seeding of a cell preparation onto a prefabricated scaffold. As a consequence, constructs are composed from the bottom to the top, the opposite of the usual scaffold based top bottom approach. Secondly, it is a form of “additive manufacturing,” where objects are built by addiction of subsequent, overlaying layers, differently from standard “subtractive methods” where the process starts from an initial block from which material is removed to reach the desired shape and size. Finally, it is a “rapid prototyping” technology allowing the fabrication of custom-made products by means of computer-assisted technologies. The process starts from patient’s medical images acquired with noninvasive techniques such as magnetic resonance imaging and computerized tomography. A recent example of this possibility is a study by Ding et al. who designed and fabricated a biphasic scaffold, consisting of PLA/PGA and poly-ε-PCL/hydroxyapatite. The construct was then utilized for the regeneration of goat femoral head. Computer-aided design and manufacturing (CAD/CAM) technology was employed to fabricate the scaffold into the desired shape and structure. Chondrocytes and bone marrow MSCs were seeded into the scaffolds for cartilage and bone regeneration, respectively. After 10 weeks, goat femoral heads were successfully regenerated. The bioprinted objects were similar, in shape and size, to native goat femoral heads. In particular, it was appreciable a smooth, continuous, avascular, and homogeneous cartilage layer on the surface and a stiff bone-like tissue underneath. Additionally, histological examination showed typical features of both native tissue, with a well-integrated osteochondral interface [20].
Despite all materials that are still under investigation, hydrogels are the most commonly used due to their swelling and lubricating properties similar to those of the native tissue and to the ability promote the chondrogenic phenotype of the encapsulated cells [20].
Bioprinting technology is really appealing for cartilage engineering since it possesses several advantages over conventional techniques, such as a reduced time required to build objects, an increased versatility and the possibility to reproduce geometrically complex structures, and graft customization. For these reasons, research and patient expectations are very high. For example, one of the most exciting possible developments of bioprinting is the possibility of in situ printing, i.e., the production of an engineered tissue directly into the defect. However, it has to be underlined that this technology is still in its infancy and needs to face many challenges, including technical, logistical, financial, and legislative problems.
7.2.2 Cells
The choice of the cell population that best allows reaching cartilage regeneration is still a challenge and nothing has been identified yet as the best. In particular, an ideal cell component should be:
Viable
Easily available and accessible
Easy to manipulate
Non-immunogenic
Non-tumorigenic
Phenotypically stable
Responsive to bioactive factors
The cell source can be autologous, avoiding immune response issues and disease transmission or allogenic to eliminate donor-site morbidity and to maximize availability. Cells can be utilized alone or in combination with a scaffold and/or biological or mechanical stimuli. They can be ex vivo expanded prior to implantation or not [21].
Chondrocytes and stem cells are the most widely used cell population for tissue articular cartilage engineering [9].
7.2.2.1 Chondrocytes
Chondrocytes were the first and most obvious choice for cartilage tissue engineering applications since they are found in the native tissue. In particular, the ACT approach by Brittberg et al. consisted in the implantation of a cell suspension composed by previously ex vivo expanded articular chondrocytes [15]. The expansion phase was needed because the limited number of chondrocytes in cartilage was not sufficient to fill clinically relevant defects. However, the use of passaged cells revealed to be problematic, since chondrocytes grown in monolayer cultures lose their differentiated phenotype and become dedifferentiated cells which synthesize molecules normally found in fibroblasts, like collagen types I, III, and V and versican. Therefore, researches have been directed towards the development of procedures using suitable biomimetic scaffolds which act as carriers for the implantable cells, maintaining at the meantime phenotype stability [22].
It has been shown indeed that seeding of chondrocytes onto collagen or HA scaffolds maintained cell viability and morphology, and promoted the production of articular cartilage ECM markers. Our group performed several studies testing a type of HA esterified-based scaffold. In the study by Grigolo et al., human chondrocytes demonstrated to be able to re-acquire the original phenotype, which they had lost in monolayer culture, by re-expressing collagen type II and aggrecan mRNAs and re-synthesizing the ECM corresponding proteins. Moreover, an ultrastructural analysis, performed by the FEISEM (Field emission in lens scanning electron microscopy) technique, showed that the cells were able to completely colonize and interact with the scaffold, by modifying their ultrastructural features in terms of shape, membrane specialization, and collagen spatial orientation [23]. In a subsequent study, the same group showed the ability of chondrocytes grown within such a scaffold to downregulate the production of some catabolic factors, such as MMP-1, MMP-13, and nitric oxide, in comparison with monolayer conditions. Apoptosis showed a decrease as well, result that was consistent with the fact that nitric oxide is one of the molecules that triggers cartilage apoptosis and breakdown via activating MMPs activity [24].
In recent times, cartilage tissue engineering interest has focused also on the possibility to treat early cartilage lesions in OA patients. To this end, Cavallo et al. compared chondrocytes from healthy donors and from OA patients for their capacity to re-express native ECM molecules, once seeded onto the already tested HA-based scaffold. Results indicated that normal chondrocytes behaved the same as OA ones in terms of cartilage markers enhancement and catabolic factors downregulation. These data are important since contributed to justify the use of tissue engineering therapeutical approach also in patients with OA [25].
In vitro chondrocyte ability to re-acquire their native phenotype, when seeded onto collagen-derived biomaterial scaffolds, has been demonstrated as well. In particular, Grigolo and collaborators tested an equine collagen fibrils membrane [26]; Roseti et al. evaluated a pig collagen types I and III scaffold displaying a bilayer structure, with a porous side and compact a one. The porous, rough side can sustain cell growth. The compact, smooth side is cell occlusive, preventing diffusion into the synovial fluid, and also protecting from mechanical impacts [27].
7.2.2.2 Stem Cells
Bone marrow stem cells have elicited significant interest in cartilage tissue engineering as an alternative to chondrocytes [28]. This is due to various reasons: the multi-lineage differentiation potential, including chondrogenesis, when cultured under defined conditions; the ease of isolation and ex vivo expansion; a very low tendency to dedifferentiate in monolayer culture, the ability to migrate to sites of tissue injury, and the immunomodulatory properties.
In a work by our group, we tested the ability of human bone marrow-derived MSCs, seeded onto the same HA scaffold used for chondrocytes experiments, to differentiate in chondrogenic sense. Transforming growth factor β (TGF-β) was utilized in the culture medium to enhance chondrocyte phenotypic differentiation. Results indicated that cells were able to express cartilage typical marker, downregulating, at the same time fibrocartilage ones. This was not really a surprise since HA is highly abundant in the mesenchyme of the developing embryo. Hence, we hypothesized that the biomaterial itself contributed to the recapitulation of the events occurring during cartilage maturation, providing not only structural but also biological support to reparative processes [29].
The multi-differentiation potential of MSCs enables their use to develop constructs containing more than one cell type. An example is the possibility to create gradient scaffolds mimicking the complex osteochondral compartment. In fact, in such conditions, MSCs have the possibility to differentiate towards the chondrogenic or osteogenic phenotype, depending on the layer structure, composition, and orientation. The works by Grigolo et al. and Manferdini et al. investigated a novel nanocomposite (type-I collagen and magnesium-enriched hydroxyapatite), porous, biomimetic gradient scaffold mimicking two or three different layers of the native osteochondral structure: cartilage, tidemark and osteochondral bone. In particular, both papers showed the ability of such scaffolds to support the growth and differentiation of expanded human MSCs towards chondrogenic or osteogenic phenotype, depending on the layer of seeding. Safranin O staining, collagen type II, and proteoglycans immunostaining confirmed that chondrogenic differentiation was specifically induced only in the superficial layer mimicking the cartilaginous structure. Conversely, von Kossa staining, osteocalcin, and osteopontin immunostaining confirmed that osteogenic differentiation occurred on both the other two layers resembling the tidemark and the subchondral bone, respectively [30, 31].
Recently, adipose-derived stem cells (ASCs) have raised attention for their similarities with bone marrow ones, in relation to morphology and immunologic phenotype. This cell source seems to display further advantages over bone marrow one. In fact, adipose tissue is more abundant and easier to obtain. Moreover, cell frequency and proliferation rate are higher than in the medullary stroma. These characteristics make ASCs a good candidate for tissue engineering applications [3].
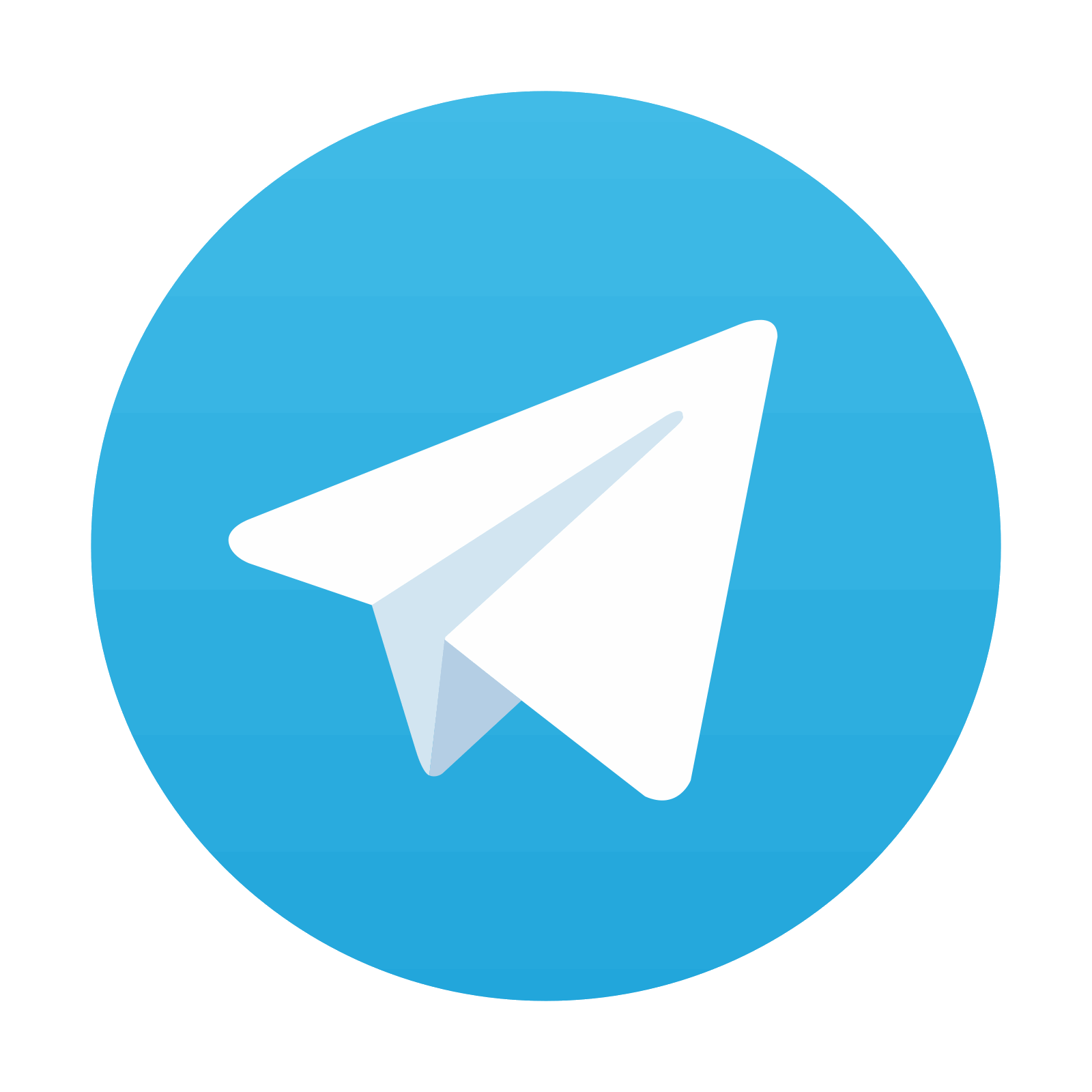
Stay updated, free articles. Join our Telegram channel
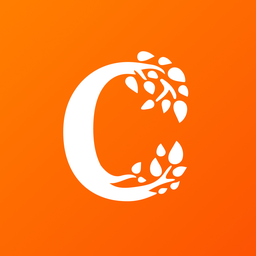
Full access? Get Clinical Tree
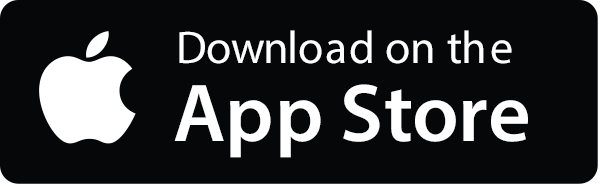
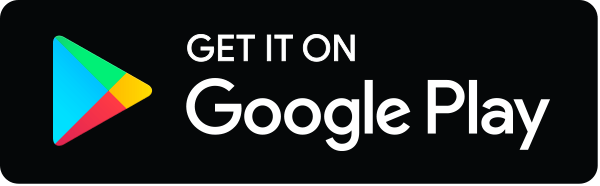
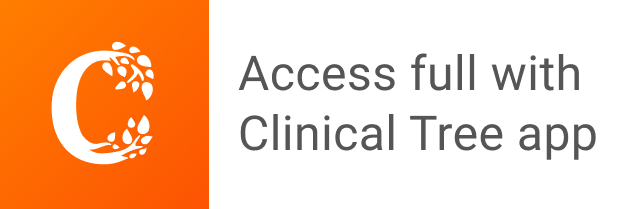