the effects of the gene product are limited specifically to the population of modified cells, the chance of adverse response from unintended diffusion to a nontarget tissue is eliminated. On the downside, overexpression of various transcription factors essential for chondrogenic differentiation and bone formation, such as RUNX2 and SOX9, has been shown to induce adverse skeletal phenotypes5 and tumorigenic activation.6 From a technical standpoint, for procedures involving direct in vivo gene delivery, for an intracellular gene product to induce a meaningful response at the tissue level or organ level, a large proportion of the resident cell population must be genetically modified by the vector. Regardless of vector type, functional in vivo gene delivery of this magnitude is an extremely challenging undertaking, even with viral systems in small laboratory animals. Further, because efficacy is a function of the number and locations of the cell populations modified by the vector (rather than total protein expression), exhaustive marker studies are required to assess and optimize dosing relative to the density and distribution of transduced cell populations in the target environment.
packaging of the vector DNA containing the cis-acting recognition sequences. The resulting recombinant viral particles can infect and transduce target cells but can only replicate in the complementing cell line. Removal of viral coding sequences from the vector genome precludes their expression in transduced cell populations, which reduces their immunogenicity and prolongs transgene expression (discussed later). As each viral vector is unique, with different genomes, host-cell range (tropism), and molecular mechanisms for transduction, the key to a successful platform involves tailoring the vector and expression cassette to the therapeutic needs of the target disease. The following sections describe the salient features of the most common viral vector systems in clinical gene therapy: adenovirus, adeno-associated virus (AAV), and retrovirus (gamma retrovirus [γ-retrovirus] and lentivirus) (Table 1).
preferred vector system for human protocols involving in vivo gene delivery.
TABLE 1 Properties of the Main Classes of Vectors Used for Gene Therapy | ||||||||||||||||||||||||||||||||||||||||
---|---|---|---|---|---|---|---|---|---|---|---|---|---|---|---|---|---|---|---|---|---|---|---|---|---|---|---|---|---|---|---|---|---|---|---|---|---|---|---|---|
|
immune responses, and, depending on the biology of the target cell, can support long-term expression of therapeutic gene products.
was linked to a scaffold and subsequently implanted into critical-size bone defects in a canine model.35 It was theorized that local progenitor cells would infiltrate the matrix, acquire the plasmid, and express the transgene to stimulate local osteogenesis and enhance repair of critical size defects. Unfortunately, the early reports of efficacy have not been widely reproducible. Attaching or incorporating exogenous genetic material into a scaffold reduces its bioavailability, such that the already inefficient process of DNA uptake becomes even more so. The problem with nonviral gene transfer is not a matter of controlled release but inefficient uptake. Newer iterations of the gene-activated matrix concept include viral vectors.
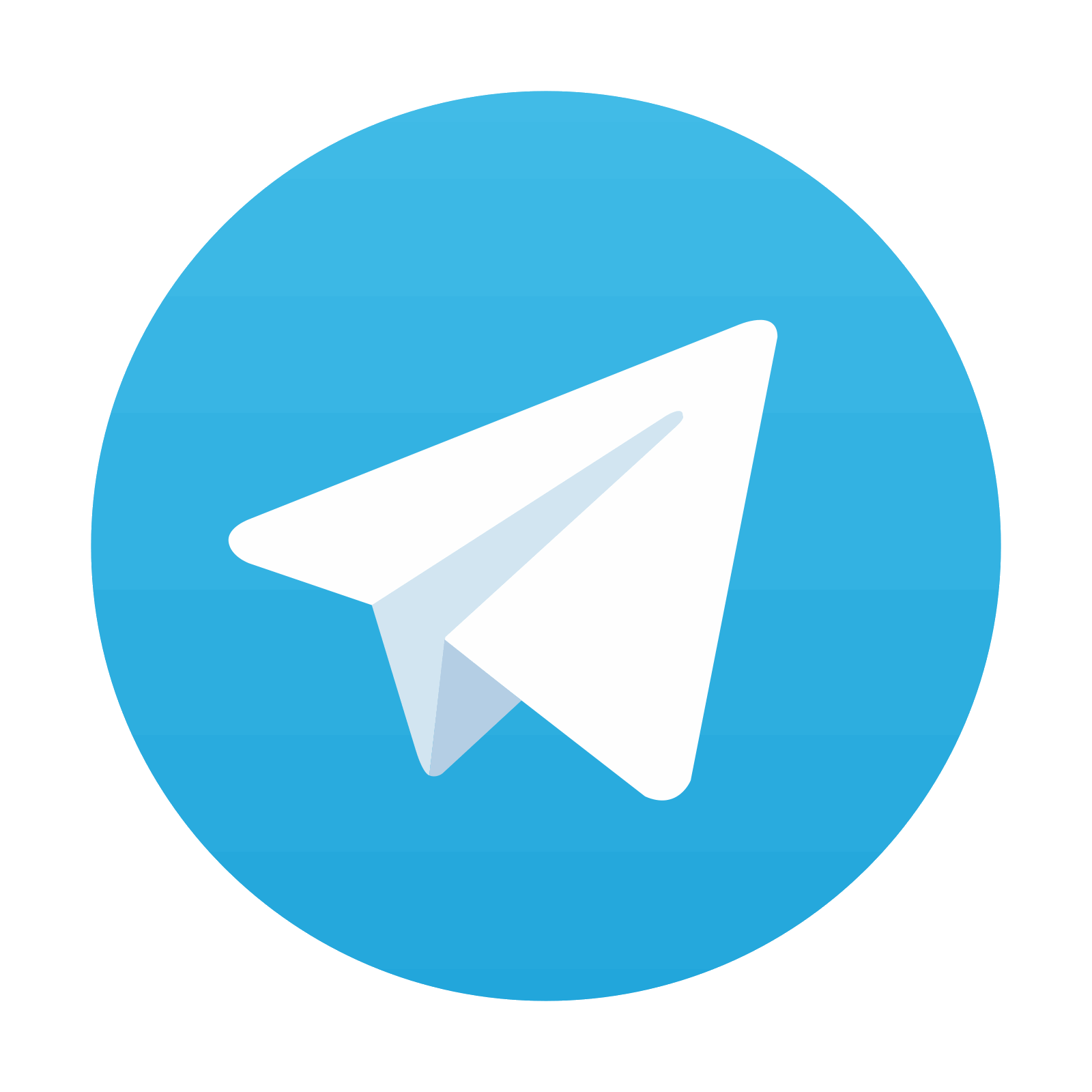
Stay updated, free articles. Join our Telegram channel
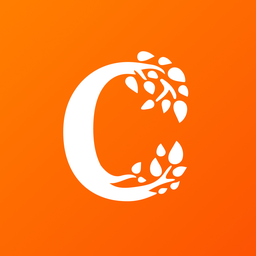
Full access? Get Clinical Tree
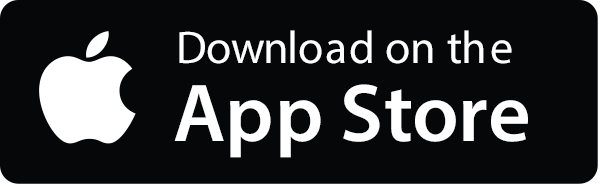
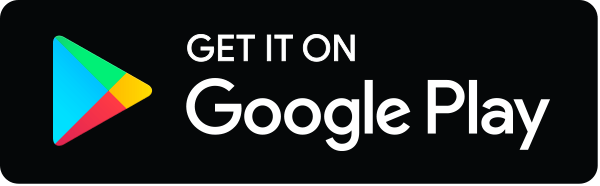