Fig. 2.1
The human vertebral column from (a) median-sagittal, (b) ventral, and (c) in a cross-sectional view of a cervical segment. Note the increasing shift between spinal segments and in the vertebrae. 1 posterior median sulcus, 2 dorsal horn, 3 dorsal column, 4 posterior median septum, 5 dorsal horn, 6 posterolateral column, 7 anterolateral column, 8 ventral horn, 9 posterior gray commissure, 10 central canal, 11 anterior gray commissure, 12 ventral (white) commissure, 13 anterior column, 14 anterior median fissure, 15 ventral root, 16 posterior spinal arteries, 17 posterior radicular artery, 18 lateral spinal artery, 19 anterior radicular artery, 20 anterior spinal artery (c) (adapted with permission from [79])
A longitudinal fissure along the spinal cord is termed the ventral (anterior) median fissure (Fig. 2.1c). The spinal cord’s ventral white commissure (Fig. 2.1c) forms its floor. The ventral median fissure has a corresponding counterpart at the dorsal side, the shallower posterior (dorsal) median sulcus (Fig. 2.1c). From there, the posterior median septum, which is made up of pial tissue, extends to the central gray matter. A typical anatomical feature of spinal cord cross sections is the “butterfly-shaped” form of the central gray matter, which has two distinct regions, the dorsal and ventral horns (Fig. 2.1c). Of note, only in the thoracic and upper lumbar spinal cord, a third horn is visible, the (intermedio)lateral horn. This region contains neurons that belong to the autonomic nervous system. The thoracic lateral horn specifically contains preganglionic sympathetic neurons. Across all segments, the central connection of the gray matter (anterior gray commissure and intermediate gray matter, respectively [79, 120]), surrounds the central canal, which is lined by ependymal cells and contains cerebrospinal fluid.
The human spinal cord is subdivided into a total of 31 segments: 8 cervical, 12 thoracic, 5 lumbar, 5 sacral, and 1 coccygeal (outlined in Fig. 2.1a). From each segment a pair of dorsal and ventral roots originates, which are composed of individual dorsal and ventral rootlets and together exit the spinal cord at the corresponding anterolateral and posterolateral depression, respectively. This point of exit (fila radicularia) is a continuity along the length of the spinal cord. The dorsal roots contain the dorsal root (spinal) ganglia, which are located within the intervertebral foramina (Fig. 2.1b). They are composed of pseudounipolar neurons, which give rise to a single axon with a bifurcation into a central and peripheral process. At the level of dorsal root ganglia, the dorsal and ventral roots of the spinal cord unite into a short spinal nerve. Of note, the nomenclature of spinal roots differs from the spinal canal segments. A segment is defined as the section of spinal cord from which a spinal nerve pair (left and right) extends toward the periphery. Consequently, the spinal cord segments do not necessarily correspond to those of the spinal canal due to the above-described developmental ascension of the spinal cord. As a consequence, the spinal roots have to descend further down to then exit the column at the appropriate intervertebral foramen (rostral for C1–C7 roots, caudal for all others). As a result, the definitive spinal cord ends at L1 with the conus medullaris (Fig. 2.1a, b), while the remaining bundle of lumbar, sacral, and coccygeal fibers further extend as the cauda equina down to Co1 (Fig. 2.1a, b).
2.1.1 Meninges of the Spinal Cord
As is typical for the CNS, three layers of meninges surround the spinal cord: (1) the superficial dura mater, (2) the arachnoid mater (intermediate), and (3) the inner pia mater. The pia mater is the origin of the denticulate ligament, an elastic structure that tethers the pia and the dura mater along the entire spinal cord. It is an essential part of the supporting structure for the cord in the spinal canal and provides stability. Three presumptive spaces appear within this sheathing of the spinal cord: the epidural, subdural, and subarachnoid space. Moving from the outside in, the epidural space is external of the dura mater and contains mostly loose connective tissue, epidural adipose tissue, the spinal nerve roots, and the internal vertebral venous plexus (see Sect. 2.1.2). In its rostrocaudal orientation, the epidural space diminishes at the foramen magnum (here, the spinal dura mater merges with the endosteal dura of the cranium) and at the sacral hiatus. In the cervical and thoracic regions, the epidural space is filled with a large basivertebral vein.
Classic textbook anatomy highlights the subdural space as a true anatomical structure between the dura mater and arachnoid. However, as discussed by Watson and colleagues [120] based on data obtained by Reina, Vandenabeele, Haines, and others, this is most likely not the case [40, 94, 118]. This “space” is more likely a preparation artifact.
Finally, the subarachnoid space lies between the arachnoid and the pia mater. It contains cerebrospinal fluid and a loose network structure of collagen fibers and fibroblasts, the arachnoid trabeculae [40]. This trabecular structure connects the arachnoid to the pia mater and further contains leptomeningeal cells traversing the space and thus creating the so-called intermediate leptomeningeal layer, which closely resembles the cranial arachnoid trabecular network [76]. Overall, the subarachnoid space extends from the cranium down to the S2 vertebra. Functionally, the subarachnoidal space plays an important role for cerebrospinal fluid drainage [91], which is mostly accomplished by arachnoid villi extending into the subarachnoid space.
2.1.2 Vasculature of the Spinal Cord
Apart from the usual functional aspects of gas and nutrient exchange, blood flow in the CNS is fundamentally important for clearance of metabolic heat. Thus, it is only logical that during embryonic development, angiogenesis and neurogenesis go hand in hand, supporting each other also by using similar signaling pathways [102, 113]. Examples of growth factors that support both angiogenesis and neurogenesis include vascular endothelial growth factor (VEGF), members of the fibroblast growth factor (FGF) family, and brain-derived neurotrophic factor (BDNF), among others (reviewed in [93]).
2.1.2.1 Arteries
The main arterial supply of the spinal cord is provided by posterior (dorsal), lateral, and anterior (ventral) vessels that span the entire cord length (Fig. 2.1c, see also [120]). The anterior spinal artery is located directly at the indentation of the anterior median fissure (Fig. 2.1c). In contrast, two posterior spinal arteries are located just ventral of the dorsal root entrance to the spinal cord, and the lateral spinal arteries can be observed somewhat halfway between the dorsal and ventral roots (Fig. 2.1c). Various transverse arteries extend from this arterial transverse anastomotic circle into the spinal cord and predominantly branch in the gray matter. The origin can be variable, but usually the anterior spinal artery is a derivate of two branches of the vertebral arteries at the level of the pyramidal decussation. The posterior spinal arteries either derive directly from the vertebral artery in the neck region or stem from the posterior inferior cerebellar artery. The posterior spinal arteries also receive blood from segmental arteries (ascending cervical artery, profound cervical artery, posterior intercostal artery, and lumbar arteries). The most prominent supporting artery is the artery of Adamkiewicz, which supplies the thoracolumbar and sacral spinal cord. In most cases it originates from the abdominal aorta approximately at T9, traverses the intervertebral foramen, and eventually anastomoses with the anterior spinal artery. Radicular arteries enter the spinal canal alongside the spinal nerves in the intervertebral foramina, then branch outside the dura mater, and follow the dorsal and ventral roots, respectively, to enter the spinal cord (Fig. 2.1c). Within the gray and white matter of the spinal cord, an intricate capillary network is formed and is significantly more elaborated in the gray matter. It was discovered in the mid-1940s that this imbalance of microvascularization mostly correlates with the number and density of synapses with high levels of mitochondria, of which there are many more in the gray than white matter [103]. Furthermore, some regions also seem to contain more capillaries than others, for example, the corticospinal tract contains about twice the amount of capillaries than the cuneate fasciculus [129].
2.1.2.2 Veins
Along the surface of the spinal cord, an elaborate venous plexus can be observed. Similar to the arterial layout, an anterior and posterior spinal vein spans the longitudinal axis of the spinal cord but can show significant caliber variations. Both the anterior and posterior veins anastomose via a network of smaller veins that circumvent the spinal cord. Especially at the cervical and lumbar enlargements, where the majority of spinal nerves innervating the extremities exit the cord, a larger venous transverse anastomotic circle can be found.
2.2 Development of the Spinal Cord
2.2.1 The Neural Plate and Neural Tube
The first significant step during vertebrate nervous system development is the achievement of neural identity from dorsal ectodermal cells positioned along the midline of the gastrulating embryo. Subsequently, the neural plate will form and, along its two major axes (anterior-posterior and dorsal-ventral), it supports the generation of all neural cell types that will ultimately shape the mature CNS.
The CNS derives entirely from ectoderm. Its earliest derivatives include the neural plate and the neural crest. The neural plate is a somewhat oval-shaped region with elevated epithelial cells rostral to the primitive pit (Fig. 2.2a). Beginning with Carnegie stage 6 (day 18pc), the neural plate can be distinguished from the surrounding ectoderm in the anterior germinal disc and is wider there than at its caudal end. The neural crest and the embryo’s placodes develop in a band of cells directly adjacent to the neural plate. These cells are in direct contact with the surrounding epidermal ectoderm.


Fig. 2.2
Embryonic development of the (a) neural plate and (b) neural tube, dorsal view. At Carnegie stage 6 (day 18pc), the neural plate can be distinguished from the surrounding ectoderm and is induced due to an inhibition of epidermis formation, which in turn is driven by signals that derive from the primitive node. Within 24 h of the first appearance of the neural plate, folds appear at its respective edges, thereby creating the neural fold along the midline. At Carnegie stage 9 (20 days pc), fusion of the opposing neural folds and subsequent formation of the neural tube occurs at the hindbrain – spinal cord junction. The two open ends after neural fold closure are the neuropores. They remain temporarily open (modified from [101, 120])
The neural plate is induced due to an inhibition of epidermis formation, which in turn is driven by signals that derive from the primitive node at the cranial end of the embryo (Fig. 2.2a), [101]. Within 24 h of the first appearance of the neural plate, folds appear at its respective edges, thereby creating the neural fold along the embryo’s midline (Fig. 2.2a, b).
Several factors contribute to the complex event of axial patterning and neurulation – the latter describing the event of embryonic folding not only along the midline but also in a dorsoventral orientation to ultimately close and form the neural tube. Cell intercalation into the embryonic midline results in a narrowing along the medial-lateral axis (convergence) and simultaneous rostrocaudal lengthening (extension) [50]. Specifically, the caudalizing event is driven by signaling molecules of the fibroblast growth factor (FGF) family, while the rostralizing event relies heavily on retinoic acid signals [56].
The other directional folding event along the dorsoventral axis is carried by bone morphogenic proteins (BMPs) for the dorsalizing orientation and sonic hedgehog (SHH) for the major ventralizing factor of this event [127]. Both factors significantly contribute to so-called hinge point (HP) formation [108]. Median and dorsolateral HPs are required for correct neural tube folding and closure, and lack of correct signaling results in the emergence of a number of neural tube closure defects along the embryo, which, depending on the position of the defect, can range from anencephaly to craniorachischisis, lumbosacral spina bifida, or spinal dysraphism and encephalocele [18, 37].
In humans, the next major time point in the development of the spinal cord is at around Carnegie stage 9 (20 days pc). Here, fusion of the opposing neural folds and subsequent formation of the neural tube occur at the hindbrain – spinal cord junction. This event requires several days to be completed and is coordinated simultaneously both at the rostral and caudal ends [82]. After closure of the neural folds, both ends temporarily remain open and are referred to as neuropores (Fig. 2.2b). When by 28 days pc both neuropores have been closed, the rostral neural tube initiates brain vesicle development, while the caudal pore is triggered to form the primitive spinal cord. This process of neural tube closure and extension of the spinal cord to S4/S5 is termed primary neurulation, with the secondary neurulation describing the event during which more caudal levels of the spinal cord are generated via connection and fusion of mesodermal cells. These cells then epithelize and subsequently merge with the rest of the tube [101].
2.2.2 Derivatives of the Neural Crest
Cells that emerge along the edge of the neural folds during the above-described process form what is termed the neural crest. They reside along the entire length of the neural tube, and while those that migrate along a dorsolateral pathway give rise to a number of neural cell populations later in life, those that transform into sensory ganglia are of distinct significance for spinal cord development: they are the origin of all dorsal root ganglia (DRG). Morphologically, the somata of these neurons remain in place, but the cells project a peripheral process to innervate somatic or visceral regions, while a central projection extends into the forming dorsal horn of the spinal cord. The extension of the peripheral projections heavily depends on appropriate trophic factor-mediated guidance and survival cues provided by the respective target sites. At the same time, a solid balance with repulsion events contributes to process pathfinding. The notochord, ventral spinal cord, and dermomyotome of the developing embryo have putative chemorepulsive effects on the extending peripheral DRG processes. Masuda and Shiga suggested members of the semaphorin family along with chondroitin sulfate proteoglycans be included among factors secreted by the notochord and dermomyotome, respectively, while the chemorepulsive carriers secreted by the ventral spine remain elusive [61].
2.2.3 Derivatives of the Alar and Basal Plates
A fundamental step during spinal cord development is the formation of the regions that will later form sensory and motor areas, respectively. A cross section through a 6-week-old human embryo is depicted in Fig. 2.3. Here, the roof and floor plates as well as a ventricular zone of the developing embryo are separated clearly. They are surrounded by neuroepithelial cells that produce a pseudostratified wall to the neural tube lumen. In this region, a number of cells with similar fates come to lie close to each other and ultimately perform cell-type-specific coupling, which depends on both chemical and bioelectrical signaling [1, 8], thus building the mantle layer between the ventricular zone and the marginal zone. The mantle layer, which resides around the primitive spinal cord, arises from multiple neuroepithelial cell divisions. These then lead to the subsequent emergence of primitive nerve cells and the accumulation of postmitotic neuroblasts beneath the external limiting membrane of the neural tube. The mantle layer will become the gray matter of the spinal cord. In turn, the marginal zone will ultimately become the white matter of the mature spinal cord.


Fig. 2.3
Cell type specification and migratory paths in the human spinal cord. At around 6 weeks of age, progenitor domains and daughter cells have been specified (inserts for basal and roof plate). In the ventral spinal cord (basal plate insert, left), five progenitor pools in the ventricular zone (p0–p3, pMN) give rise to five mature neuron populations in the mantle zone (V0–V3, MN). In the dorsal spinal cord (alar plate insert, right), six progenitor domains (dp1–dp6) give rise to six early-born interneurons (dl1–dl6) and two late-born interneurons (dlLA, dlLB). Cells and processes in the center graphic depict migratory routes of neuroblasts (see text for details). 1 spinal canal, 2 roof plate, 3 alar plate, 4 basal plate, 5 floor plate, 6 matrix zone, 7 mantle zone (later gray matter), 8 marginal zone (later white matter), 9 dorsal root, 10 dorsal root ganglion, 11 ventral root, 12 spinal nerve, 13 ependymal cell (guides radial migration of neuroblasts), 14 arcuate fibers (guide tangential migration of neuroblasts), 15 motoneurons, FP floor plate, RP roof plate (adapted from [79, 133])
Within the mantle layer, two distinct, paired regions can be observed: the dorsal thickening is referred to as the alar plate (future sensory areas of the spinal cord) and the ventral thickening is the basal plate (future motor areas of the spinal cord). Notably, the cells that reside outside of the roof and floor plates will ultimately serve as dorsal and ventral white commissures that facilitate the crossing of axons in the mature spinal cord.
In terms of cellular derivatives, the alar and basal plates and their induction to produce the cell types of the spinal cord have been investigated mostly in animal models. However, it is reasonable to assume similar mechanisms for human spinal cord development, as many of the signaling molecules and guidance factors along with their gene regulation are highly conserved among vertebrate species. While the roof plate is most likely initiated by signaling from the overlying ectoderm [16, 59], the floor plate is induced by axial mesoderm [2, 72]. Once established, the floor plate has been shown to generate a SHH gradient, which in turn induces the production of distinct pools of progenitors in the neuroepithelial ventricular zone ([13, 133], Fig. 2.3 left panel). Likewise, the roof plate and its external ectoderm, by generating a BMP gradient that is also reciprocal to the floor plate’s SHH gradient, produces six progenitor domains, which in turn generate six distinct early-born and two late-born dorsal interneuron cell populations [133] (Fig. 2.3 right panel). Like in the developing brain, the majority of neuroblasts use migration paths along radial glia cells to leave the matrix zone and reach the mantle zone (Fig. 2.3). However, not all neuroblasts follow this radial migration pattern. A subset of neuroblasts has been observed to migrate tangentially. For example, axons of some early-differentiating alar plate-derived neurons extend toward the ventral spinal cord and intersect in the floor plate. These arcuate fibers build a pathway, the so-called circumferential pathway [42], which in various species serves as both a dorsal-to-ventral [54] and ventral-to-dorsal migration path for neuroblasts [87]. Thus, it provides important commissural axon corridors that are essential for correct target innervation.
2.2.4 Cell Type Specification of the Spinal Cord
2.2.4.1 Motoneurons
All motoneurons originate from a single ventral progenitor domain (Fig. 2.3, left panel), yet strikingly, their delicate specification later allows for the coordinated movement of the large variety of muscles in the human body. For this, the positional identity of motoneurons along the spinal cord’s rostral-caudal axis is key. This position and location identity is the direct result of the concerted action of multiple signaling molecules (for review see [21, 107]).
These spatiotemporal signaling events lead to different birthdates of motoneuron populations. Early-born motoneurons migrate into the basal plate while trailing a radially oriented process that has a distinct central orientation, already aiming at its target region. Next, the emerging motoneuron develops an early axonal domain, which is distinguishable from the cell’s somatodendritic domain by expression of early axon-specific markers such as scaffolding proteins for the evolving axon initial segment [53]. Finally, the axons will traverse the marginal zone and emerge from the ventral surface of the cord, thus forming the ventral roots of the spinal cord. Likewise, dendrites will branch into the newly forming neuropil of the ventral horn and gray matter.
Ventral horn neurons arise from five distinct columnar subpopulations, of which four are interneuronal and one is motoneuronal (Fig. 2.3, left panel). The latter will produce three columns of neurons: (1) lateral motoneurons positioned at the cervical and lumbosacral enlargement of the spinal cord will later innervate the musculature of the extremities, (2) medial motoneurons that can be found throughout the entire spinal cord will later innervate axial muscles, and (3) visceral motoneurons that arise from an intermediolateral column in the thoracic and cranial lumbar segments. At 8–10 weeks pc in humans, motoneurons will segregate into specific somatic motor columns, a process which depends both on genetic and epigenetic regulation [19]. Francius and Clotman most recently published a comprehensive review on motoneuron specification and diversity [34].
As already mentioned above, target-finding and long-term survival of motoneurons depends predominantly on specific guidance- and survival cues. In this context, far more motoneurons are produced than will ultimately survive and innervate target sites. Several signaling molecule families are involved in this important elimination event, for example, classic neurotrophins (BDNF, NT3, NT4), several members of the cytokine family (ciliary-derived neurotrophic factor (CNTF), leukemia inhibitory factor (LIF), cardiotropin-1), several TGF-β family members (glial-derived neurotrophic factor (GDNF), neurturin, artemin, persephin), insulin-like growth factors IGF-I and IGF-II, and members of the fibroblast growth factor family (FGF-1, FGF-2, and FGF-5) (for review see [58, 106]).
2.2.4.2 Interneurons
Interneurons are born both in the areas composed of, and directly adjacent to, the dorsal roof plate as well as the ventral floor plate (Fig. 2.3). The dorsal spinal cord establishes six distinct progenitor pools (dp1–dp6), which in turn give rise to six early-born (dI1–dI6) and two late-born interneuronal subtypes (dILA and dILB) that differ by birthdate, relative dorsoventral position, and gene expression profiles [133]. Interneurons in the ventricular zone close to the floor plate emerge from the above mentioned four progenitor domains p0–p03 that result in mature interneuronal subtypes V0–V3 (Fig. 2.3, also refer to Sect. 2.5.3.1). Interestingly, many of these early subtypes have been found to develop into commissural interneurons projecting to the contralateral side of the spinal cord. In the mature CNS, these commissural interneurons are essential for left-right locomotor coordination and represent a major component of central pattern generators (CPGs) that play a fundamental role for the rhythmic, coordinated movement of limbs and trunk [117]. CPGs will be discussed in more detail in Sect. 2.5. It is important to keep in mind that while the nomenclature for interneuron subtypes may seem simple with four major ventral classes V0–V3 and six dorsal classes, respectively, recent data actually identified several subsets within each class depending on the expression of specific sets of transcription factors [35].
2.2.4.3 Glia
Glial subtypes of the early spinal cord include radial glia, oligodendrocytes, astrocytes, and ependymal cells. Generally, gliogenesis occurs after the brief phase of neurogenesis, and astrocytes, which develop in large parts from radial glia [6, 64], emerge before oligodendrocytes, which later are the sole source for CNS myelin. Notably, in humans, oligodendrocyte precursors first reside in the ventral ventricular zone close to the floor plate and in the direct neighborhood of a motoneuron domain [41]. In fact, data from genetic studies suggest that oligodendrocytes and motoneurons share a common ancestral progenitor [60, 109]. In contrast, ventral interneurons seem to share a common ancestor with different astrocyte subtypes that ultimately settle in various gray and white matter areas depending on their origin [97, 115]. Oligodendrocytes are sequentially produced in three distinct waves. First, ventral precursors from the motoneuron domain arise and migrate along the entire neural tube. As with motoneuron specification, the development of these cells is SHH mediated and is repressed by dorsally secreted BMPs and WNT proteins. The second wave occurs later in the fetal phase. Here, progenitors located in the dorsal spinal cord develop from dp3–dp6 interneuron domains. Finally, the third wave is characteristically initiated after birth. The origin of these cells remains elusive [70, 97].
Ependymal cells eventually come to line the central canal of the spinal cord but are absent from other spinal structures. They guide radially migrating neuroblasts during development (Fig. 2.3). Last but not least, microglia appear in the human spinal cord around 9 weeks pc and undergo major infiltration of the tissues from 16 weeks pc on [96]. They most likely colonize the developing spinal cord along with the emerging vascularization of the tissue [96].
2.2.5 Emergence of Ascending and Descending Spinal Tracts
The earliest time point at which discernible ascending (spinocerebellar) neurons appear in humans is 10 weeks pc [17]. In a similar time window, by 13 weeks pc, the lateral corticospinal tract in humans has reached the caudal medulla oblongata. Just 2 weeks later, the pyramidal decussation is completed (reviewed in [112]). Next, the cervical spinal cord is reached between 14 and 16 weeks pc, and then a brief stop in further progression can be observed. The corticospinal tract invades the more caudal regions of the spinal cord at a later stage of development: 17 weeks pc for the low thoracic spinal cord, 27 weeks pc for the lumbosacral spinal cord. The early contact between descending axons of the corticospinal tract and corresponding synaptic targets in the spinal cord represents an important event for proper structural and functional maturation of the spinal cord. The maturation of spinal motor centers requires neuronal activity provided by the aforementioned contact [31]. This activity-dependent pattern for correct tract formation is especially important, considering that the developmental hallmark of initial exuberant axonal growth with subsequent substantial axon loss depends largely on neuronal activity and growth factors (whose expression can also be activity dependent). Hence, descending inputs are essential for the induction of plasticity and appropriate circuit formation [110]. In fact, recent data suggest that different motor systems can interact during development to drive each other’s adult specification [123]. The authors suggest that the developing rubrospinal system is under activity-dependent regulation by the corticospinal system for establishing mature rubrospinal connections and a corresponding red nucleus motor map.
2.2.6 Myelination of Spinal Cord Tracts
Contrary to many mammals where myelination is part of the postnatal developmental period, first myelination of axons in the human can be observed in the early fetal spinal cord (e.g., less than 16 weeks pc shown by [78]). However, the majority of myelination cannot be seen until the second trimester. A distinct temporal order of myelinating events has been described previously. The earliest structure to myelinate is the medial longitudinal fasciculus (20 weeks pc), with the corticospinal tract being the last, still undergoing myelination at birth [121]. The same authors also described a spatiotemporal gradient of myelination, in which the three major protein classes myelin basic protein (MBP), proteolipid protein (PLP), and myelin-associated glycoprotein (MAG) appear along an anteroposterior and rostrocaudal gradient, with MBP emerging first. On a molecular level, oligodendrocyte progenitors – after migration and arrival at the correct location – undergo terminal differentiation into so-called premyelinating oligodendrocytes. This event follows a rostrocaudal gradient in the spinal cord [11]. Their terminal differentiation then includes the actual process of myelination, which requires physical contact to the axon that is to be myelinated. Again all these steps underlie intricate molecular regulation that depends on a number of developmental genes and factors (for a detailed review, see [70]).
2.3 Cytoarchitecture and Pathways of the Spinal Cord
2.3.1 Spinal Cord Gray Matter
Based on a fundamental anatomical nomenclature for the cat spinal cord gray matter first published by Rexed [95], a similar laminar cytoarchitecture of the gray matter also applies to humans [105]. An overview is provided in Table 2.1. The dorsal horn includes laminae I–VI, while laminae V–X reside in the base of the dorsal horn and the central region of the ventral horn (Fig. 2.4). However, not all laminae can be clearly distinguished from each other. Laminae I–VIII span the entire length of the spinal cord, although their size can vary significantly from one segment to the next. Of note, lamina IX cannot be understood as one section in the classical sense, but rather as motoneuron pools interspersed within laminae VII and VIII (Fig. 2.4).

Table 2.1
Laminae and nuclei of the spinal cord
Region | Nuclei | Laminae |
---|---|---|
Dorsal horn | Marginal zone | I |
Substantia gelatinosa | II | |
Nucleus proprius | III, IV | |
Neck of dorsal horn | V | |
Base of dorsal horn | VI | |
Intermediate zone | Stilling-Clarke’s nucleus, intermediolateral nucleus | VII |
Ventral horn | Commissural nucleus | VIII |
Motor nuclei | IX | |
Gray matter around central canal | Grisea centralis | X |

Fig. 2.4
Cytoarchitecture of the spinal cord gray matter. Left side: Lamina of the gray matter after the nomenclature of Rexed indicated by roman numerals (laminae I through X). Right side: 1 tract of Lissauer, 2 marginal cells (lamina I), 3 substantia gelatinosa (laminae II + III), 4 nucleus proprius (lamina IV), 5 intermediate zone, 6 intermediate zone (laminae V–VIII), 7 lateral motor column (lamina IX), 8 ground bundles (fasciculi proprii), 9 medial motor column (lamina IX), 10 ventral root (modified with permission from [79])
Lamina I (zona marginalis, substantia spongiosa) has a reticular appearance and is composed of predominantly fusiform neurons (marginal cells). It borders with the tract of Lissauer, the region, where the dorsal horn contacts the pial surface of the spinal cord. It is visible as a band of unmyelinated or sparely myelinated afferent fibers also known as the posterolateral tract and contains axons projecting toward central regions, carrying nociceptive and thermoreceptive information. Neurons in lamina I are important for nociception and thermoreception and are the principal site for termination of nociceptive afferents. They use mainly glutamate, substance P, and calcitonin gene-related peptide (CGRP) as well as other peptides as transmitters. Lamina I neurons can also respond to mechanical stimulation from Aβ-fibers [124]. Interneurons in lamina I modulate nociception and signal predominantly via opioids, γ-aminobutyric acid (GABA), and glycine.
Like lamina I, lamina II (substantia gelatinosa) is a major nociceptive transmission and computation center and principal site for termination of Aδ- and C-fibers. It is composed of a great number of smaller, densely packed neurons and many unmyelinated axons. Neurons in lamina II form mostly local connections, with terminal axon collaterals reaching from lamina III as deep as lamina V [55]. Lamina II neurons function mostly as interneurons, and an estimated third of these contain GABA and glycine [114]. Other neurotransmitters in lamina II interneurons include CGRP, substance P, and vasoactive intestinal polypeptide (VIP).
Neurons in laminae III and IV (nucleus proprius) are larger and less densely packed than in laminae I and II due to numerous myelinated axons that traverse this lamina. Again, a large portion of neurons here are interneurons, with dendrites that extend mostly rostrocaudally. Transmitter types include GABA, glycine, dopamine, and substance P, among others. Functionally, some neurons seem to respond to fine tactile information, while others respond to rather strong, coarse pressure application. In the upper cervical segments, the medial region of lamina IV builds the internal basilar nucleus, a caudal extension of the cuneate nucleus [7].
Lamina V is the site of convergence between somatic and visceral input. It can be further divided into a medial part (ascending projection neurons) and a lateral part (preganglionic neurons in the lateral horn). The latter contains large somata, intercalated with thick myelinated axon tracts. In the human, this lamina cannot be clearly separated from lamina VI [104]. Dendrites in lamina V extend mostly in a dorsoventral orientation and can reach into laminae II and VII, respectively. Neurons in lamina V receive afferent input from the skin, musculature, and visceral organs with mixed modalities (mechanosensory, nociceptive, chemoreceptive). The major neurotransmitters present are GABA, dopamine, glycine, and substance P, among others.
Lamina VI is the deepest layer of the dorsal horn. As mentioned before, this lamina is almost indistinguishable from laminae V and VII, only visible in the cervical and lumbar enlargements. Similar to lamina V, it is also divided into a medial and lateral zone. Afferent input comes from collaterals of Aα-fibers (from muscle spindles). Lamina VI also receives input from cutaneous and nociceptive fibers. The majority of lamina VI neurons are either propriospinal neurons or interneurons. The latter are more local in their projection, many of them innervating ventral horn motoneurons. Hence, the major function of lamina VI interneurons seems to be the control of reflex pathways.
Lamina VII comprises most of the intermediate zone. The cell population in lamina VII is evenly sized and spaced, and the majority of cells are premotor interneurons projecting to the large motoneurons of lamina IX. In fact, lamina VII is a motor computation hub in that descending motor pathways control spinal motoneurons by synaptic transmission via interneurons in lamina VII. It is assumed that lamina VII interneurons are typically prewired to execute all aspects of a voluntary movement [120]. The modular arrangement of these interneuron-to-motoneuron connections thus provides a very potent way for the brain to execute directed voluntary movements. Indeed, the topographical arrangement of motoneuron columns matches the distribution of interneurons in lamina VII, with those modulating proximal musculature being placed more medial, and those driving distal movements being located more laterally.
Lamina VII contains three distinct nuclei: (1) the nucleus dorsalis (Stilling-Clarke, (C8) T1–L2/L3; see Sect. 2.4), with afferent innervation from proprioceptive fibers from muscles and joints; (2) the central cervical nucleus (C1–C4), with afferent input from the head and neck musculature, the vertebral joints, and the vestibular nuclei, which all coordinate head and neck movement; and (3) the intermediomedial nucleus (T1–L3), a part of the autonomic cell columns in lamina VII (cholinergic neurons). The latter are interconnected with sympathetic cells from the intermediolateral nucleus (lateral in C8–L3). These cells are preganglionic, visceroefferent neurons of the sympathetic and parasympathetic systems with input from supraspinal centers in the hypothalamus, pons, and medulla oblongata. Importantly, these nuclei also receive afferent visceral input. Both the intermediolateral and medial nuclei are connected with each other and with the contralateral side. Also located in lamina VII (lateral part) are the parasympathetic sacral nuclei (S2–S4), whose neurons innervate the large intestine, the urethral and anal sphincter, the reproductive organs, and the urinary bladder (see Sect. 2.4).
Neurons in lamina VIII are heterogeneous in size and shape at different levels along the spinal cord and their dendrites project mostly in the dorsoventral orientation. Dorsal dendrites, while not crossing the midline, are extended toward lamina VII and the ventral gray commissure. The majority of cells are GABAergic with input from propriospinal afferents and from supraspinal, vestibular, and reticulospinal afferents. Neurons of lamina VIII have a central role in the coordination of motor activity. For example, the left-right coordination of movement seems to be controlled by lamina VIII neurons [80]. Also, the majority of neurons with projections in the long propriospinal pathways that connect the lumbar and cervical enlargements are located in lamina VII, and they play a fundamental role for the coordination of front and hind limb movement [120]. Further details are discussed in Sect. 2.5.
Lamina IX is not a true lamina, but a set of columns in laminae VII and VIII and along the edge of X. It is the major site for large α-motoneurons (innervate striate musculature) and smaller γ-motoneurons (innervate muscle spindles) in the spinal cord. They are surrounded by a specific class of interneurons, so-called Renshaw cells, which provide recurrent inhibition for motoneurons and thus build an essential negative feedback mechanism for motoneuron activity. Motoneurons in lamina IX are organized in columns, which differ in size and number depending on the segmental level. Generally, four distinct columns are separated:
- 1.
Medial group: Innervation of axial musculature; divided into the anteromedial nucleus (C1–Co1) and posteromedial nucleus (C1, T1–L2).
- 2.
Lateral group: Innervation of intercostal and peritoneal wall musculature; clear differentiation into separate nuclei in the region of the cervical and lumbar enlargements with the anterior and anterolateral nuclei (C5–C8, L2–S1), the posterolateral nucleus (C5–C8, L2–S2), and the retroposterolateral nucleus (C8–T1, S1–S3). These innervate the musculature of the extremities.
- 3.
Central group: Only visible in specific segments. From C3–C5, contains the nucleus of the phrenic nerve (innervation of the diaphragm); from C1–C5, contains the nucleus of the accessory nerve (ultimately becomes one origin of the accessory nerve after exiting the spinal cord and ascending in the subarachnoidal space through the foramen magnum).
- 4.
Onuf’s nucleus: In S1–S3, with motoneurons of the pudendal nerve, innervates the striate muscles of the urethra sphincter and rectum.
Lamina X (central gray of the spinal cord) extends along the entire spinal cord and encompasses the region around the central canal. Neurons in this lamina are smaller than those from the surrounding lamina VII and are more densely packed. Neurons of the central autonomic area are located here. Cells in lamina X receive input from C-fibers and Aδ-fibers of both somatic and visceral origin. Likewise, lamina X functions in both nociception and visceroception (including visceral pain and mechanoreception). Axons from lamina X project into laminae V, VI, and VII, among other targets in central regions of the brain.
2.3.2 Spinal Cord White Matter
As outlined in Sect. 2.1, the horns of the gray matter divide the surrounding white matter into three columns: a dorsal (posterior), a lateral, and a ventral (anterior). Yet boundaries between these parts are not necessarily distinct and clear. Within the white matter, ascending and descending pathways are distinguished from each other and will be discussed in the following sections (summarized in Table 2.2).
Table 2.2
Summary of the most important ascending and descending long tracts of the spinal cord
System/pathway | Origin | Decussation | Level of termination | Function |
---|---|---|---|---|
Posterior column–medial lemniscal pathways | ||||
Cuneate fasciculus | Peripheral afferents, above T6 | Uncrossed | Ipsilateral cuneate nucleus | Proprioception, vibration sense, fine touch |
Gracile fasciculus | Peripheral afferents, legs, and lower trunk | Uncrossed | Ipsilateral gracile nucleus | Proprioception, vibration sense, fine touch |
Anterolateral system | ||||
Spinothalamic tract (lateral and anterior) | Especially laminae I–II, also V | Ventral commissure of the spinal cord at the level of the originating segment | Mostly VPL of the thalamus | Discriminative aspects of nociception + thermoreception (location, intensity), tactile input (nondiscriminative) |
Spinoreticular tract (sometimes seen as part of spinothalamic tract) | Laminae VI–VIII | Predominantly ipsilateral, some fibers in anterior white commissure | Medullary-pontine reticular formation with projection to intralaminar thalamic nuclei | Emotional + arousal aspects of nociception |
Spinomesencephalic tract | Laminae I and V | Anterior white commissure | Periaqueductal gray (midbrain), superior colliculus, midbrain raphe nuclei, parabrachial nucleus | Emotional component of nociception |
Spinotectal tract | Laminae I and V | Anterior white commissure | Deep layers of superior colliculus | Reflex reaction after nociceptive stimuli (turning of eyes/head/upper body toward stimulus) |
Spinohypothalamic tract | Lamina II | Anterior white commissure | Hypothalamus | Autonomic + reflex responses (e.g., endocrine, cardiovascular), nociceptive input |
Cerebellar input system | ||||
Cuneocerebellar tract | Accessory or lateral cuneate nucleus (input from C2–T5) | Uncrossed | Ipsilateral anterior lobe of cerebellum via restiform body | Proprioceptive input from ipsilateral neck + upper limb |
Rostral spinocerebellar tract | Dorsal horn (C4–C8), lamina VII | Uncrossed | Cerebellum | Proprioceptive input from ipsilateral head + upper limb |
Dorsal (posterior) spinocerebellar tract | Dorsal nucleus of Clarke (C8–L2/L3), lamina VII | Uncrossed | Ipsilateral cerebellar vermis | Proprioceptive input from ipsilateral lower trunk + lower limbs |
Ventral (anterior) spinocerebellar tract | Dorsal horn; laminae V–VII of L, S, and Co levels; L3–L5 anterolateral border of anterior horn (spinal border cells) | Anterior white commissure, then back to ipsilateral in the superior cerebellar peduncle | Ipsilateral cerebellar vermis | Proprioceptive input from ipsilateral lower trunk + lower limbs |
Lateral motor system | ||||
Lateral corticospinal tract | Primary motor cortex, other frontal, parietal regions | Pyramidal decussation (cervicomedullary junction) | Entire cord (cervical and lumbar enlargements) | Movement of contralateral limbs |
Rubrospinal tract | Nucleus ruber (magnocellular) | Ventral tegmental decussation (midbrain) | Cervical segments of the cord | Movement of contralateral upper limbs (flexor muscles) |
Medial motor system | ||||
Anterior corticospinal tract | Primary motor cortex, supplementary motor area | Anterior white commissure | Cervical, upper thoracic segments of the cord | Control of biaxial and girdle musculature |
Vestibulospinal tract (VST) | Medial VST: medial and inferior vestibular nuclei; lateral VST: lateral vestibular nucleus | Bilateral Ipsilateral | Medial VST: cervical, upper thoracic cord; lateral VST: entire cord | Antigravity muscles, Medial VST: head/neck positioning; lateral VST: balance |
Reticulospinal tracts | Pontine and medullary reticular formation, medial + lateral | Uncrossed | Entire cord, laminae VII + VIII (medial) and VII + IX (lateral) | Maintenance of posture, gait-related movements, modulation of muscle tone |
Tectospinal tract | Superior colliculus | Dorsal tegmental decussation (midbrain) | Cervical cord | Head/eye coordination (uncertain in humans) |
Overall, the ascending and descending tracts can be subdivided further into functional groups (Figs. 2.5 and 2.6). In the ascending orientation, we find (1) the posterior column-medial lemniscal system, which relays sensory information on vibration, proprioception, and fine touch (via the cuneate and gracile fasciculus), (2) the anterolateral system, which transmits nociceptive, thermoreceptive, and crude touch information (via the anterior and lateral spinothalamic tracts, spinoreticular tract, etc.), and (3) the cerebellar input system, which is responsible for proprioceptive sensibility of the upper and lower limbs (composed of the dorsal spinocerebellar tract, cuneocerebellar tract, and smaller tracts such as ventral and rostral spinocerebellar tracts).
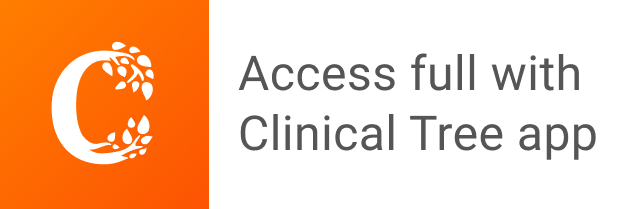