Fig. 21.1
Axonal degeneration, regeneration, and sprouting after spinal cord injury. (a) Injured non-regenerating axons display dystrophic retraction bulbs and Wallerian degeneration of the distal axon segment. Axon regeneration can occur into and across the lesion site or around the lesion. (b) Sprouting of injured and non-injured spared axons can be observed above or below the lesion site
Axons in the peripheral nervous system (PNS) retain the ability to regenerate after injury throughout adulthood, whereas CNS axons do not. For a long time, the dogma that adult CNS neurons are unable to regenerate held true, as there was no evidence of CNS axon growth even into permissive peripheral nerve tissue implanted into the brain of rabbits [148]. However, subsequent studies demonstrated that the central branches of dorsal root ganglion (DRG) neurons are capable of intraspinal regenerative sprouting after partial denervation of the spinal cord in cats [157]. CNS regeneration remained controversial for several decades [115] until experiments by Aguayo and colleagues provided final proof that transected CNS axons can regrow into bridges of growth-permissive peripheral nerves implanted into the rodent spinal cord [1, 218]. This led to the hypothesis that CNS neurons are not generally incapable of regenerating but are rather hampered by the inhibitory nature of the CNS environment encouraging approaches to counteract this inhibition [41]. Subsequent studies have suggested that overcoming environmental cues alone is not sufficient to obtain robust CNS regeneration: CNS neurons display a lower growth potential than peripheral nervous system (PNS) neurons even on a permissive substrate in vitro, and this is associated with the limited activation of cell intrinsic programs after an axotomy [48].
Thus, current data suggest that the difference in the regenerative capacity of PNS and CNS is not only due to extrinsic factors acting primarily at the injured axon tip but also due to neuron-intrinsic differences. Extrinsic factors include the lack of positive growth signals present in PNS but not in the CNS, like laminin and Schwann cell-secreted factors, and the presence of negative cues accumulating at the site of injury in the CNS but not in the PNS including myelin-derived inhibitors, ephrins, semaphorins, and proteoglycans. Therefore, to address extrinsic influences, provision of a more permissive substrate, neutralization of the inhibitory CNS environment, and growth factor delivery have been investigated as a means to enhance CNS regeneration. More recent investigations have also focused on the intrinsic regenerative potential, which is regulated by a wide range of cellular processes including membrane and cytoskeletal reorganization, metabolic changes, axonal transport, local protein translation and signaling cascades, and the activation of a pro-regenerative genetic program.
21.2 Extrinsic Factors in Axonal Regeneration
21.2.1 Inhibitors of Axonal Regeneration and Sprouting
Axonal regeneration and neuroplasticity are limited by neurite growth-inhibitory molecules in the CNS, in particular after injury. The cellular and molecular responses that contribute to the inhibitory environment after CNS injury are now much better understood, and means to overcome growth inhibition have moved from in vitro neurite growth assays and preclinical animal models to clinical studies. Major components of the inhibitory environment after CNS injury include the fibroglial scar within and around the lesion, proteoglycans in the extracellular matrix, myelin-based inhibitors in particular in white matter, and several soluble repulsive factors.
21.2.1.1 The Fibroglial Scar
After SCI, a fibroglial scar consisting of different cells and extracellular matrix is formed within and around the lesion epicenter. Historically, the astrocytic scar was thought to be the main impediment for axonal regeneration. Indeed, hypertrophic astrocytes dominate in the lesion penumbra, but the lesion core contains a heterogeneous mix of cells, including NG2+ glia/oligodendrocyte precursor cells, meningeal and/or vascular fibroblasts, pericytes, ependymal cells, and phagocytic macrophages [245]. Disruption of the meninges is associated with infiltration of meningeal fibroblasts, which form a fibrotic scar and contribute to the inhibitory environment by promoting astrocytic reactivity [278] and by expressing repulsive guidance molecules [101]. However, the glial scar also stabilizes the injured parenchyma by reestablishing its physical and chemical integrity, closing the blood–brain barrier, reducing the infiltration of non-CNS cells, and limiting possible infections [35]. Ablating hypertrophic astrocytes or impeding their reorganization into a sealing cellular barrier is deleterious and results in invasion of inflammatory cells beyond the lesion, an increase in lesion volume, and functional deterioration [75, 117, 196, 279]. Thus, the classical view of the glial scar as deleterious in CNS injuries has clearly been refuted. However, at the same time the fibroglial scar constitutes a physical and molecular obstacle to regenerating axons. Several experimental strategies have attempted to modify the growth-inhibitory fibroglial scar. These include means to prevent the deposition of collagenous matrix [233], blocking TGF-ß [2, 58, 59], which contributes to astrocyte gliosis, making astroglia more permissive for growth by overexpressing cell surface molecules such as L1 or PSA-N-CAM [45, 71, 228, 290] or cell transplantation (discussed below). Besides the physical and cellular barrier generated by the fibroglial scar, factors produced in and around the lesion site actively prevent axonal growth into and beyond a lesion in the injured spinal cord. The most important inhibitory factors are discussed below.
21.2.1.2 Proteoglycans
One class of molecules present in the extracellular matrix of the glial scar actively inhibiting axon outgrowth are chondroitin sulfate proteoglycans (CSPGs). CSPGs consisting of a protein core and glycan side chains are strongly upregulated at sites of CNS injury [132, 133, 175]. In the 1990s, in vitro studies established a clear link between astrocytes, CSPG secretion and inhibition of neurite outgrowth. Astrocyte lines producing a nonpermissive matrix were poor promoters or inhibitors of axon growth, which was reversed by treatment with inhibitors of proteoglycan synthesis [248–250]. In vivo studies demonstrated that upregulation and distribution of CSPGs in the scar tissue after SCI contribute to the failure of axon regeneration [61, 62]. CSPGs are also present throughout the CNS around cell soma and dendrites in perineuronal nets and thereby limit plasticity in areas of the CNS not directly affected by injury [171, 245]. Digestion of glycosaminoglycan (GAG) side chains of CSPGs with the bacterial enzyme chondroitinase ABC (ChABC) can attenuate its inhibitory activity, and the first evidence that the enzyme is effective in vivo in animals with spinal cord contusion injuries suggested that CSPG digestion could be of therapeutic value [153]. Indeed, intrathecal delivery of ChABC after cervical dorsal column lesions was shown to promote regeneration of ascending sensory and descending corticospinal projections in rodents, electrophysiological improvements, and partial recovery of sensorimotor function [30]. Subsequent studies have confirmed the beneficial effects of ChABC treatment after SCI, including enhanced regeneration of lesioned axons [37, 38, 244, 289], enhanced sprouting and connectivity of spared pathways [11, 30, 171, 253], immunomodulatory mechanisms [66], and neuroprotection [42, 43].
Both somatic and autonomic motor recovery was observed after digestion of CSPGs [30, 38, 152]. Most studies conducted to date were done in rodent models of SCI, with few studies in larger animals such as cats [261] and squirrel monkeys [27]. However, studies in larger animals are likely needed to assess the safety and efficacy of ChABC treatment and to better estimate required dosage and develop suitable means of delivery. Studies with ChABC in dogs are currently ongoing (http://vetmed.iastate.edu/vmc/small-animal/clinical-trials/chondroitinase-clinical-trial-0). In recent years, combined delivery of ChABC with other approaches, such as immunomodulation [110], cell and peripheral nerve transplantation [4, 44, 86, 152, 267], delivery of neurotrophic factors [95, 124, 137], antibodies against myelin-based inhibitors (anti-Nogo-A) [291], or rehabilitation [276], has shown that such combinatory treatments are even more effective.
Although beneficial effects of ChABC have been observed and validated across several independent laboratories in different animal models, clinical translation remains challenging. ChABC rapidly loses its enzymatic activity at body temperature, and therefore repeated injections are needed, with increased risk of infections due to the invasive route of application. This issue has been partially addressed by thermostabilization of the enzyme, delivery within hydrogel scaffolds, generation of ChABC-secreting cell lines, and delivery by viral vectors [13, 125, 137, 223]. However, long-term delivery by viral gene transfer might pose additional problems, as the consequences of prolonged or permanent CSPG degradation are unknown.
Recently, receptors mediating the inhibitory activity of CSPGs have been identified and include the protein tyrosine phosphatase σ (PTPσ), the phosphatase leukocyte common antigen related (LAR), and the Nogo receptors 1 and 3 (NgR) [65, 81, 242]. The overlap in receptors for Nogo (see below) and CSPGs [65] indicates that shared mechanisms for neurite growth inhibition by myelin-associated inhibitors and CSPGs exist. Peptides blocking LAR or PTPσ receptors preventing CSPG binding increase serotonergic axon sprouting after SCI in mice, and LAR knockout mice show better functional recovery after SCI [81, 147]. Intracellularly, CSPGs inhibit neurite growth by signaling through the Rho GTPase/ROCK pathway [64, 177, 186], and inactivation of Rho by C3 transferase is one means to modulate responses to growth-inhibitory CSPGs [64]. A formulation of C3 transferase (Cethrin) that is cell permeable and blocks Rho activation [161] has moved to clinical trials [77]. The phase I/IIa study with a small number of patients does not allow conclusions about treatment effects, but a trend toward larger motor recovery was evident [176]. Ibuprofen has also been suggested as a potential candidate to modulate Rho activity, and several reports have indicated an effect of high doses of ibuprofen in animal models of SCI [143].
21.2.1.3 Other Inhibitory Molecules
In addition to CSPGs, several other classes of potent growth-inhibitory molecules are upregulated after SCI and are expressed by cells within and around the lesion site including semaphorins, ephrins, and Eph receptor tyrosine kinase family members, Wnts and netrins [101, 103, 193, 198].
Members of the Eph protein family are upregulated for several weeks post-injury in the rodent spinal cord [52, 127]. EphA and EphB3 receptor expression is increased in glia and neurons [185, 284]; ephrinB2 can be detected on reactive CNS astrocytes, and its receptor EphB2 is present on infiltrating fibroblasts, suggesting a close interaction between the different cell types [33, 74]. EphrinB3 expressed by adult oligodendrocytes has also been identified as a strong inhibitor of axon regeneration [16]. Soluble inhibitors of Eph4A signaling (ephrin-5A-Fc or Eph4A-Fc) or EphA4-blocking peptides have shown to promote some improvement in axon growth [74, 104].
Meningeal fibroblasts, contributing to the lesion scar, are known to express semaphorins [201], and pharmacological inhibition of Sema3A resulted in improved regeneration and/or preservation of injured axons, Schwann cell-mediated myelination, a decrease in apoptosis, and better functional recovery after SCI in rats [136]. Taken together, these findings indicate that blocking of semaphorins or of Eph/ephrin signaling could represent a viable option to promote regeneration after SCI.
21.2.1.4 Myelin-Associated Inhibitors
The concept that adult CNS myelin contains growth-inhibitory components was proposed more than 30 years ago. This was based on the fact that CNS axons can regenerate in peripheral nerve transplants [57, 218], while PNS axons extend only poorly on CNS tissue [235]. In fact, even PNS neurons display more limited neurite extension on CNS myelin than on PNS myelin [76, 236].
The proteins underlying the contact-mediated inhibition of neurite growth were isolated, and an antibody (IN-1) against the inhibitory myelin component was shown to partially neutralize its growth-inhibitory effect [41]. Based on this information, the gene was identified and cloned and is now known as Nogo-A [46, 107, 207]. In parallel, other myelin-associated components have been identified that have potent inhibitory activity on neurite outgrowth [97]. These include myelin-associated glycoprotein (MAG), oligodendrocyte myelin glycoprotein (OMgp), and CNS myelin lipid sulfatide [156, 178, 277, 285]. All myelin inhibitors signal via a common receptor (NgR) in a complex with Lingo-1 and TROY or p75. Activation of NgR induces axonal cytoskeletal rearrangements and retrograde transport to the cell body, where it exerts further inhibitory functions via a signaling pathway involving the small Rho GTPase, RhoA, and Rho-associated kinase (ROCK) [68, 199].
Although MAG and OMgp represent a nonpermissive growth substrate, experimental ablation of MAG did not abolish the inhibitory effect of CNS myelin in vitro and in vivo [12]. Equally, ablation of Nogo-A or its receptor in mice resulted in contradictory findings promoting regeneration or having no effect on axon growth [36, 67, 140, 150, 151, 246, 292, 293]. One potential explanation for the limited effects in knockout animals is the presence of multiple other inhibitors; thus deletion or neutralization of a single inhibitory component or receptor complex can only have limited effects. As MAG, OMgp, and Nogo-A seem to signal through the same Nogo receptors (NgR), and overlap in inhibitory signaling from CSPGs exists, intracellular signals downstream from the receptor might have a higher likelihood of being therapeutically active.
In one of the first studies demonstrating an effect of antibodies against Nogo-A, intrathecal infusions of the monoclonal antibody IN-1 raised against two inhibitory antigens (NI-35 and NI-250, now known as Nogo-A) [41] enhanced sprouting of injured corticospinal axons in rats [234]. Several studies confirmed some of these findings across different animal species, including nonhuman primates. Nogo-A was targeted in vivo by different anti-Nogo antibodies, the NgR-blocking peptide NEP1-40 or a soluble Nogo receptor fusion protein (NgR-Fc) [84, 87, 90–92, 106, 140, 155, 189, 255]. In rodents, treatment with anti-Nogo-A led to enhanced regenerative sprouting, midline crossing of unlesioned contralateral CST fibers after unilateral pyramidotomy, some regeneration and faster recovery of sensory function, and locomotion [155, 210, 263]. Similarly, anti-Nogo-A-treated nonhuman primates subjected to unilateral spinal cord transection at the cervical or thoracic level showed corticospinal axon sprouting and recovery of manual dexterity of the ipsilesional hand, although this recovery was discussed controversially [84, 92, 270].
Based on the preclinical evidence, a humanized, recombinant anti-Nogo-A antibody was produced by Novartis for clinical application. Toxicological studies were performed in rodents and primates. Phase I of the anti-Nogo-A clinical trial, sponsored by Novartis, was started in 2006 within the EMSCI network of spinal cord centers (European Multicenter Study about Spinal Cord Injury, EMSCI; http://clinicaltrials.gov/ct2/show/NCT00406016). Furthermore, anti-Nogo-A (ozanezumab) was tested in ALS patients, as Nogo-A has been proposed as an early diagnostic biomarker of ALS (http://clinicaltrials.gov/ct2/show/NCT00875446). Ozanezumab was well tolerated and only six adverse events were reported, none of which was related to the drug. Immunohistochemical analyses of frozen sections of muscle biopsies from ALS patients showed co-localization of ozanezumab and Nogo-A [181]. Results from the trial in SCI patients have not been published to date. Although both clinical trials were successfully completed, a phase II trial is only now being initiated in Europe.
21.2.2 Neurotrophic Factors
Besides the inhibitory environment in the injured spinal cord, the absence of permissive growth substrates at the lesion, insufficient stimulation of axon growth, and a lack of guidance (chemotropic or physical) for regenerating axons contribute to the regenerative failure. During development and after injury in the PNS, neurotrophic factors play an important role in neuronal survival, axonal growth, and target innervation. Several families of trophic factors including members of the neurotrophin family (nerve growth factor (NGF), brain-derived neurotrophic factor (BDNF), neurotrophin-3 (NT-3), neurotrophin-4/5 (NT-4/5)), GDNF family ligands (glial cell line-derived neurotrophic factor (GDNF), neurturin (NRTN), artemin, persephin), neuropoietic cytokines (ciliary neurotrophic factor (CNTF), leukemia inhibitory factor (LIF)), and others have therefore been investigated for their influence on neuronal survival and axon growth after SCI. In addition to infusions and injections of protein, numerous studies employed cells genetically modified to express neurotrophic factors or direct in vivo viral gene delivery to enhance survival, sprouting, and regeneration.
21.2.2.1 Effects on Neuronal Survival and Atrophy After SCI
Neuronal atrophy and cell death can be consequences of axotomy depending on the distance to the cell soma and the affected neuronal population. After SCI, most spinal projection neurons in the brain do not die but some become atrophic. In fact, the survival of injured neurons is reflected in the presence of dystrophic end bulbs of axotomized neurons, which can be found at and around the lesion site in SCI patients even over 40 years after the trauma [227]. The shrinkage of cell bodies has sometimes been mistaken for cell death, but more accurate methods for cell counts have in most cases not confirmed neuronal loss.
One of the clearest examples for SCI-induced neuronal atrophy can be observed in the red nucleus. Rubrospinal neurons shrink after cervical spinal cord lesions and BDNF delivery in the vicinity of the cell soma, either acutely or even 18 months post-injury, can prevent or reverse neuronal shrinkage [142, 144, 226]. Corticospinal neuron cell death after subcortical lesions or atrophy of CST neurons after SCI can also be amended by BDNF [31, 100, 162]. Another example is the effect of NT-3 in rescuing spinal projection neurons [29] and neurons in Clarke’s nucleus in the spinal cord [243].
21.2.2.2 Neurotrophic Factors in Regeneration and Sprouting
In addition to promoting neuronal survival, neurotrophic factors also promote axon growth during development, after PNS injuries and following neurotrophic factor delivery to the injured spinal cord. Many animal studies examining axonal responses to growth factor delivery have used grafts of cells genetically modified to overexpress neurotrophic factors. Depending on the trophic factor expressed, projections from the brain stem such as rubrospinal, raphespinal, cerulospinal, reticulospinal, and spinal projections including propriospinal, spinal motor axons, and primary sensory axons have been shown to extend into growth factor-producing cellular grafts such as fibroblasts [20, 22, 108, 109, 118, 129, 130, 179, 190, 271], Schwann cells [182, 280], bone marrow stromal cells [164, 165], olfactory ensheathing cells [39, 224, 225], neural precursor cells [40, 160], or peripheral nerve grafts [24].
Most axonal populations responding to different trophic factors (Table 21.1) densely penetrate growth factor-producing grafts except for corticospinal axons, which have only convincingly been shown to penetrate grafts of fetal spinal cord. While NT-3 delivery promotes corticospinal sprouting, growth is only observed in the host parenchyma surrounding the lesion or in the distal spinal cord if some spared projections remain [24, 47, 83, 108, 224, 294].
Table 21.1
Axonal populations responding to cellular or viral neurotrophic factor delivery
Neurotrophic factor | Responding axonal populations | References |
---|---|---|
NGF | Nociceptive sensory | |
BDNF | Rubrospinal | |
Reticulospinal | ||
Raphespinal | ||
Vestibulospinal | [130] | |
Dorsal column sensory | [164] | |
NT-3 | Dorsal column sensory | |
Corticospinal | ||
NT-4/5 | Propriospinal | [22] |
Raphaespinal | [22] | |
GDNF | Dorsal column sensory | [20] |
Nociceptive sensory | [20] | |
Propriospinal | ||
IGF-1 | Raphaespinal | [120] |
Despite the robust axonal growth of some axonal populations into a lesion site filled with neurotrophic factor-releasing cells, axons rarely exit the graft to extend into the distal host tissue. Thus, sustained cellular delivery of neurotrophic factors stimulates axon growth, but the inhospitable environment and the lack of a growth stimulus beyond the lesion site remain an obstacle for long-distance axonal bridging across the lesion site. Even if transient, regulated cellular delivery of the neurotrophin BDNF is used, axons do not extend beyond the graft, although transient growth factor delivery is sufficient to sustain regenerated axons in the lesion [21]. Only in the presence of a growth stimulus distal to the cell graft, axons can exit the lesion. Using virus-based in vivo gene delivery (lentivirus or adeno associated virus (AAV)), gradients of NT-3, BDNF, or GDNF within the distal host tissue can be generated, allowing for bridging axonal regeneration of dorsal column sensory axons, reticulospinal axons, and propriospinal axons, respectively [5, 63, 134, 166, 167, 260]. Successive regulated gradients of neurotrophic factors might further increase the distance covered by regenerating axons.
In addition to regeneration of injured axons, neurotrophic factors can also promote sprouting and structural rearrangement of spared connections, as well as changes at the cellular and synaptic level (reviewed in [28, 173, 282]. Collateral sprouting is functionally highly relevant and contributes to spontaneous neurological recovery in animal models of SCI and most likely in patients with incomplete SCI. Such naturally occurring neural rearrangements that have been extensively investigated in rodents represent an attractive target to enhance functional recovery [7, 10, 53, 281]. Collateral sprouting of spared projections after neurotrophic factor delivery has been shown for corticospinal axons in response to NT-3 delivery [47, 294] and for reticulospinal projections and serotonergic axons [231] after BDNF delivery to name a few.
21.2.2.3 Adverse Effects and Difficulties in Clinical Translation
While there is strong evidence for the pro-regenerative activity of growth factors in the injured spinal cord, potential adverse effects pose challenges for the translation of findings from animal models. This is partially due to the widespread distribution of receptors but also depends on the duration and the amount of growth factor delivery. Intraventricular and intrathecal infusion via osmotic mini-pumps lead to widespread distribution of growth factors, and long-term delivery can result in Schwann cell proliferation, weight loss, and nociceptive fiber sprouting as demonstrated for NGF infusions. Intraparenchymal in vivo gene delivery or grafting of genetically modified cells can partially overcome these issues. However, a reliable means to turn off neurotrophic factor expression in virus-transduced cells is needed as long-term growth factor expression results in graft expansion likely by Schwann cell proliferation [20, 22]. In addition, sustained high levels of growth factors within a lesion site will not promote axon extension across a lesion site. Only few studies have examined regulatable neurotrophic factor expression indicating that regenerated axons are at least partially sustained once growth factor expression is turned off [21, 122]. High doses of BDNF delivered by viral vectors are needed for axons to regenerate across a lesion site but can also induce spasticity-like symptoms and hyperreflexia in rats after cervical or sacral SCI [85, 167]. Taken together, only a very localized, transient, and possibly cell-restricted elevation of growth factors might be suitable for clinical translation.
21.3 Neuron-Intrinsic Factors Influencing the Regenerative Capacity
The growth capacity of CNS axons declines during development as connections mature and synapses are formed (see chapter 2). This decline is accompanied by the downregulation of growth-associated genes and is also ultimately reflected in the regenerative failure of adult CNS neurons. However, based on the responses of different axonal populations to a variety of growth-promoting stimuli, differences in the regenerative capacity seem to exist in the adult spinal cord. Sensory axons seem to have the highest competence for regeneration followed by propriospinal and brain stem projections. Corticospinal neurons are most refractory to regenerative approaches. The ability of peripheral sensory neurons to mount a regenerative program after PNS injury has been a major focus in identifying genes and signaling cascades important for axon regeneration. Indeed, if injury to the central projections of dorsal root ganglion neurons in the spinal cord is preceded by a lesion in the PNS, regeneration can even be observed in the spinal cord. This so-called conditioning effect is based on the activation of regeneration-associated genes in DRG neurons and has served as a model to identify mechanisms underlying intrinsically regulated regenerative programs [191, 217]. Transcriptional and epigenetic mechanisms, local protein translation, retrograde and anterograde axonal transport, and cytoskeletal dynamics have been identified as intrinsic key regulators of axon regeneration.
21.3.1 Calcium Transients and Elevation of cAMP
When axons are injured, calcium influx into the axoplasm is one of the first consequences (see chapter 19). This transient intracellular calcium wave propagating from the injured axon to the cell soma seems to be crucial for resealing the axonal membrane, protein synthesis, cytoskeleton rearrangement, assembly of the growth cone, and the activation of intracellular signaling cascades. Axotomy-induced calcium influx leads to increased cAMP levels in neurons capable of initiating a regenerative response. Increases in cAMP levels are necessary for growth cone assembly [99] and can partially overcome myelin-associated repulsive signals in vitro and in vivo [184, 251, 252]. Injection of a cell permeable cAMP analogue (dibutyryl-cAMP; db-cAMP) into DRGs partially mimics a conditioning lesion enhancing the regeneration of injured dorsal column axons [166, 192, 208]. An alternative approach to increase cAMP levels is the administration of phosphodiesterase (PDE) inhibitors to limit the degradation of cAMP. Delivery of the PDE4 inhibitor rolipram led to enhanced regeneration, attenuation of the glial scar, and some functional recovery in rodents [194]. The pro-regenerative and neuroprotective effects of rolipram, as well as the functional improvement were also reported in contusive animal models of SCI [14, 51, 126]. However, systemic PDE inhibitors act on numerous other neuronal populations with adverse effects such as severe nausea, and changes in the expression of growth-associated genes are very transient by increasing cAMP [23]. Clearly, increases in cAMP are not solely responsible for the molecular cascades orchestrating a regenerative response.
21.3.2 Epigenetic Regulation of Regeneration-Associated Genes (RAGs) and Growth Cone Dynamics
When the calcium wave reaches the soma of DRG neurons, it leads to activation of PKCμ and export of histone deacetylase 5 (HDAC5) from the nucleus. As a consequence, increased histone acetylation contributes to the pro-regenerative transcriptional program, and local tubulin deacetylation at the axon tip by HDAC5 and HDAC6 results in increased microtubule dynamics and axon regeneration [49, 50]. HDAC5 nuclear export is followed by increased acetylation of histone 4 (H4) in the promoters of regeneration-associated genes (RAGs), which leads to their induction. Several transcription factors including Jun, KLF4, KLF5, Fos, ATF3, and Gadd45g, as well as Smad1, Sprr1, Galanin, NPY, and VIP, which have been associated with the injury-induced response and axon growth, have been found to be HDAC5 regulated [50, 80]. Independently, the histone acetyltransferase CBP/p300 regulates RAG expression via histone 3 (H3) acetylation of p53, GAP-43, and Sprr1 [96]. Thus, epigenetic mechanisms might play a key role in axon regeneration.
21.3.3 Manipulation of the Cytoskeleton
Injured PNS axons maintain stable proximal microtubuli and have dynamic microtubuli at the tip of their growth cone, whereas injured CNS axons form retraction bulbs with a disorganized network of microtubuli, a rather static structure that can persist for years after SCI [227]. After nuclear export, HDAC5-mediated deacetylation of microtubuli at the axon tip of DRG neurons increases their dynamics and reorganization. This is a prerequisite for the formation and motility of growth cones, which are necessary for growth initiation and axonal extension [49, 50]. On the other hand, selective inhibition of HDAC6 and the consequent increase in acetylated, stable microtubuli enhance survival after oxidative stress and growth of CNS neurons on nonpermissive substrate [220]. Thus, microtubule stability might have divergent effects on axon regeneration. Nevertheless, pharmacological stabilization of microtubuli can promote growth cone formation and dynamics. Moderate stabilization of microtubuli by low doses of Taxol, a cancer drug that inhibits cell division by interfering with the microtubule network, has been shown to prevent the formation of retraction bulbs, decrease axonal degeneration in vivo, and enable CNS neurons to overcome the growth-inhibitory effect of myelin in vitro [73]. In the injured rat spinal cord, intraparenchymal Taxol delivery led to a reduction of the fibrotic scar in a TGF-ß-dependent manner, decreased levels of inhibitory CSPGs at the lesion, and increased the density of serotonergic fibers distal to the lesion. Moderate functional recovery was observed in Taxol-treated animals after mild contusion injuries [116]. These data were partially reproduced in a recent replication study showing changes in the extracellular matrix composition at the lesion but no functional improvements [206]. Experiments in the optic nerve also support the conclusion that Taxol can enhance regeneration and limit scar formation after injury [239]. Using a Taxol derivative, EpothiloneB, which can cross the blood–brain barrier, further supported functional recovery with this approach [227]. Taken together, despite some convincing preclinical evidence, additional studies are needed to define the dose, time window of treatment, and maximum benefit before moving toward clinical translation.
In addition to microtubuli, actin cytoskeleton dynamics play an essential role in growth cone extension. As mentioned in the description about inhibitory molecules in axon regeneration, myelin-based inhibitors and proteoglycans exert their inhibitory effect by activation of the small GTPase Rho, which acts as an intracellular molecular switch. RhoA and related GTPases act via several intermediaries on the actin cytoskeleton leading to depolymerization of actin filaments and growth cone collapse. Thus extrinsic and intrinsic regulators of regeneration closely interact and cannot always be clearly separated.
21.3.4 Transcriptional Networks and Other Signaling Cascades
The orchestrated response of DRG neurons after PNS injury leading to the upregulation of regeneration-associated genes is not only controlled by the initial calcium wave propagating from the site of injury to the cell soma but also by the retrograde transport of locally activated injury signals containing a nuclear localization sequence [15, 183]. Among the identified candidates are activated kinases (ERK, JNK) and transcription factors like STAT3. Several gene array studies have characterized the time course and extent of gene expression changes during PNS regeneration identifying an ever-increasing set of RAGs. Because CNS neurons fail to effectively activate many of these RAGs [78], means to promote CNS axon growth by gene delivery of RAGs or by manipulating pathways that lead to the upregulation of RAGs have been explored in numerous studies. Among the first genes investigated in these studies was GAP43 [3]. In addition, transcription factors that might have more broad effects by influencing numerous other genes have been explored in this context including SMAD1 [296], CREB [94], STAT3/SOCS3 [247], ATF3 [237], c-JUN [211], and others. Among these, STAT3 and c-JUN are perhaps those with the strongest indication for a pro-regenerative effect; however none of these experiments promoted regeneration to the same degree as conditioning lesions. In vitro high-throughput screens for transcription factors that enhance or inhibit axon growth have also identified members of the Kruppel-like factor (KLF) family as potential transcriptional repressors or enhancers of axon regeneration, and KLF7 can also promote corticospinal axon regeneration and sprouting in vivo after overexpression [18, 187].
Besides transcriptional networks, regulators of translation have also been shown to be involved in the regenerative program. The activity of mTOR, a regulator of protein translation, is strongly influenced by PTEN. Elimination of the tumor suppressor gene PTEN induces robust axon growth of retinal ganglion neurons [200]. Further investigations demonstrated that mTOR activity also regulates sprouting responses of CST neurons after injury. Conditional deletion of PTEN attenuated injury-induced loss of mTOR activity in CST neurons and enhanced sprouting and regenerative growth indicating that this signaling pathway represents a promising approach to target the intrinsic regenerative capacity of neurons after SCI [56, 98, 158]. Combining PTEN deletion with the activation of the STAT3 pathway showed even more remarkable growth after an optic nerve crush injury [247, 256].
21.3.5 Cell Transplantation
The therapeutic approaches reviewed above aim to enhance regenerative axon growth and/or sprouting of injured and spared projections by targeting either extrinsic or intrinsic factors. The regenerative capacity of endogenous projections remains, however, very limited, and axons only extend for modest distances. Considering the long distance that regenerating axons need to cover in the human spinal cord, robust long-distance target reinnervation after severe spinal trauma might be utopic. Moreover, boosting axon regeneration alone does not lead to restoration of neural tissue integrity and function. Neuroregenerative approaches might therefore be more effective in combination with cell replacement strategies, which promote anatomical and functional repair. Cell transplantation should ideally (1) provide a permissive physical and cellular substrate for axon growth, (2) promote axon regeneration, (3) allow for remyelination of regenerating axons, and (4) replace neurons and glia lost due to injury.
While a number of different cell types have been used in animal models and in initial clinical trials (Table 21.2), the “ideal” cell transplant covering all these functions has not been identified. Certain neural stem cells might be closest to meeting these requirements. Because cells are complex biological systems, numerous variables influence outcomes of cell transplantation experiments such as age and gender of the donor, cell source, culturing conditions, as well as means, site, and amount of delivery. Thus, different studies using similar approaches do not always come to the same conclusion. Some of the most studied cell populations are described below.
Table 21.2
Clinical trials for neuroregeneration after SCI
Category | Substance/treatment | Target | Company/sponsor | ClinicalTrials.gov ID number |
---|---|---|---|---|
Stem cells | Embryonic stem cell-derived oligodendrocyte precursors | Remyelination | Geron Asterias Biotherapeutics | NCT01217008 NCT02302157 |
Fetal brain-derived neural stem cells | Remyelination | StemCells Inc. | NCT01321333 NCT02163876 | |
Fetal spinal cord-derived neural stem cells | Remyelination? Relay formation? | Neuralstem Inc. | NCT01772810 | |
Peripheral glia | Schwann cells | Axon growth, remyelination | University of Miami | NCT02354625 NCT02354625 |
Olfactory ensheathing cells | Remyelination Axon growth | Various investigators | NCT01231893 others not listed | |
BMSCs | Axon regeneration? | Various investigators | Too many to list | |
Inhibitors of axon regeneration | Rho inhibitor (Cethrin, BA210) | Rho | Bioaxone Biosciences | NCT00500812 |
Ibubrofen | Rho Neuroprotection Axon growth | University of Berlin | NCT02096913 | |
Anti-Nogo antibodies (ATI355) | Axon sprouting/regeneration | Novartis | NCT00406016 |
21.3.5.1 Mesenchymal Cells
Mesenchymal stromal or stem cells (MSCs) have been extensively used for transplantation into the CNS. These self-renewing/multipotent stem cells, isolated from the bone marrow, can differentiate into osteoblasts, adipocytes, and chondroblasts, as well as putative neural cells and myoblasts in vitro [128]. MSCs represent a very attractive and promising cell source for tissue repair because they can be easily obtained from autologous bone marrow, cryopreserved and expanded in a relatively short period of time [238]. In addition, cells are well tolerated, and there are no reports of adverse reactions in both autologous and allogeneic transplantations. Initial reports that MSCs can differentiate into neural cells in vitro and in vivo turned out to be incorrect and were based on cell fusion or the transient expression of a few neural markers [163, 275].
MSCs have been reported to have anti-inflammatory, neuroprotective, and pro-regenerative effects by decreasing demyelination and scar formation, promoting regeneration and guiding axons, and altering the inflammatory reaction via neurotrophic paracrine activity (reviewed in [170, 197]. Improvements of locomotor, sensory, and autonomic function, as well as reduction of neuropathic pain, were observed in many but not all studies in different animal models [154, 262]. However, benefits in well-conducted preclinical studies were modest raising the question whether an invasive transplantation procedure with potential adverse effects is justified. Nevertheless, a number of ongoing and completed clinical trials have assessed safety and potential beneficial effects of BMSC transplantation after SCI. While most of these trials only enrolled a small number of patients, and are unable to draw conclusions about clinical efficacy, no major adverse effects were observed [82, 273].
21.3.5.2 Stimulated Macrophages
Despite the potential detrimental role of the immune system in CNS injury, inflammation can also play a beneficial role after SCI. Macrophages are crucial for clearing extracellular matrix and cellular debris and secrete growth factors, thereby facilitating remyelination and axon growth. In the late 1990s, transplantation of nonactivated blood-borne macrophages after transection of the spinal cord in rodents led to enhanced regeneration and recovery of motor function [216], as well as decreased expression of the axon growth-inhibitory myelin protein MAG, increased angiogenesis, and Schwann cell infiltration [89]. In a later study, a skin biopsy was adopted as the source to activate autologous macrophages that were administered 8–9 days after a rat spinal cord contusion. This resulted in less pronounced syringomyelia and improved motor function [25].
In subsequent clinical trials, autologous macrophages activated by incubation with autologous skin biopsies were injected into the spinal cord caudal to the lesion [131, 141, 146]. In both clinical trials, transplantation was performed in ASIA impairment scale (AIS) A patients within 14 days after injury. While the macrophage cell therapy was well tolerated, no significant difference in primary outcomes (conversion from AIS A to B or C) between treated and control group was detected.
21.3.5.3 Schwann Cells and Peripheral Nerves
Schwann cells play a central role in PNS regeneration, express neurotrophic factors, provide a favorable extracellular matrix, and guide axons across a lesion after PNS injury. Equally important, Schwann cells can myelinate PNS and CNS axons, they can be harvested from peripheral nerve biopsies and can be expanded in vitro.
In animal models of SCI, peripheral nerve grafts and Schwann cells have been used for over 30 years [57, 70, 218] and were investigated in a variety of animal studies, mostly in rodents. From a translational perspective, peripheral nerve grafts have several limitations compared to Schwann cell suspension grafts. These include the difficulty of creating a suitable interphase between the host spinal cord and peripheral nerve and the potentially invasive nature of nerve grafting particularly after incomplete SCI. In contrast, in vitro cultivation of Schwann cells allows for the detailed characterization of cell properties and for the generation of a sufficiently large number of cells that can be injected and distributed with limited manipulations in the parenchyma surrounding the lesion site via cell migration. For autologous transplantation, Schwann cells are usually harvested from the sural nerve resulting in limited functional impairment.
In rodent studies, Schwann cells have been transplanted in contusion/compression, hemisection, and full transection models of SCI [34]. Although several studies demonstrated that Schwann cells promote axon regeneration, only a limited number of axons can bridge the lesion site, and regenerated axons are not able to exit the graft to extend into the host tissue [286, 288]. When administered in combinatorial approaches with neurotrophic factors, degradation of inhibitory CSPGs or increases in cAMP, more robust regeneration is usually observed [8, 44, 63, 86, 102, 137, 182, 202, 280, 287]. In thoracic and cervical contusion models, slight improvements in motor function were also demonstrated after adult rodent Schwann cell transplantation [9, 232, 259].
Only few preclinical studies with human cells have been performed. Small behavioral benefits were observed, and about 1 % of axons were estimated to bridge across the lesion for a few mm. In contrast to other axons, corticospinal axons only entered grafts for very short distances and only when combined with FGF-1 [113, 114]. Based on the preclinical evidence, three clinical studies with Schwann cell transplants in SCI patients have been conducted to date. From the first two completed studies, only limited conclusions about efficacy can be drawn, but the transplantation procedure appeared to be safe [229, 230, 295]. Supported by safety and toxicity studies in rodents, minipigs, and primates, more recently, an FDA-approved phase I study transplanting autologous Schwann cells in patients with subacute neurologically complete, thoracic SCI was initiated, which is still ongoing [112] (https://clinicaltrials.gov/ct2/show/NCT01739023).
Future clinical applications will have to cope with some challenges:
- 1.
Autologous isolation of Schwann cells requires sacrificing a peripheral nerve, and thus an alternative source would be desirable.
- 2.
Expansion of nerve-derived Schwann cells or induction of Schwann cell differentiation from other cell sources [17] takes weeks to months.
- 3.
In isolation without any additional interventions, Schwann cells seem to have only limited functional effects.
- 4.
Cell survival following transplantation is rather low similar to other transplanted cells.
Nevertheless, Schwann cells remain a reasonable treatment approach for SCI. While current clinical studies might not show meaningful clinical benefit, the results could provide the basis for future studies combining Schwann cell transplants with other treatments that have shown promise in animal models.
21.3.5.4 Olfactory Ensheathing Cells (OECs)
Olfactory ensheathing cells (OECs) were first described in the nineteenth century by Golgi [105] and Blanes [19] as specialized glial cells exclusively located in the olfactory nerve and glomerular layers, one region of the CNS where axonal regeneration is possible throughout adulthood. OECs ensheath and isolate olfactory axons from the growth-inhibitory CNS environment, enabling axonal transition from PNS to CNS, i.e., from the olfactory epithelium toward targets in the olfactory bulbs [69]. The unique axonal growth-promoting properties of OECs were confirmed in vitro and in vivo after dorsal root transections [212] as well as after complete thoracic transections [214]. In the following years, the pro-regenerative and neuroprotective effects of OECs were investigated in various rodent models of SCI in several dozen preclinical studies (reviewed in [213, 222]. These studies demonstrated that OECs do not only promote axonal regeneration in the injured CNS but also functional reconnection of injured axons, remyelination, formation of blood vessels, and reorganization of the glial scar and also display immunomodulatory properties [88, 222]. However, other studies showed only limited or no functional effects comparing OECs with Schwann cells [9, 203, 259]. This might be at least partially attributable to the source of the transplanted cells, culturing conditions, number of passages, and the site of injection [6, 213, 219].
The preclinical evidence encouraged the initiation of several clinical studies. In Australia OECs isolated from nasal biopsies were transplanted in patients with chronic SCI. These studies confirmed the feasibility and safety of the approach. While functional improvements were not detected, the studies with three to six patients were too small to draw definitive conclusions [79, 169]. Two subsequent clinical trials reported functional improvements [215, 257], but again, a limited number of patients were included. Larger clinical trials are needed to confirm the safety and efficacy of OECs for the treatment of SCI.
21.3.5.5 Neural Stem Cells: Remyelination and Relays
The cells described above have demonstrated some beneficial effects after SCI by increasing neuroprotection, enhancing remyelination, or promoting regeneration. However, none of these cells are native to the CNS and can therefore only partially restore and not replace all functions normally performed by CNS neurons and glia. CNS neural stem cells that can generate appropriate neuronal and glial phenotypes might be the most efficient way for functional restoration and tissue replacement. CNS astrocytes and oligodendrocytes can provide physical, trophic, and metabolic support and remyelinate spared axons, while neurons might serve as a relay station between axotomized neurons and their targets thereby bridging the lesion site (Fig. 21.2). Neuronal relays that forward ascending and descending information across a lesion would make long-distance axon regeneration across a lesion site unnecessary. Due to their potential to differentiate into all three neural lineages (i.e., neurons, astrocytes, and oligodendrocytes), neural stem cells (NSCs) or neuronal- and glial-restricted precursors have gained substantial interest in recent years.


Fig. 21.2
Cell transplantation to promote axonal regeneration or neuronal relay formation. (a) Cellular transplants can provide a continuous substrate for axonal growth and promote regeneration of injured axons beyond the lesion site to restore connectivity. (b) Transplanted neural stem cells (green) or immature neurons that differentiate and mature can receive input from injured axons and reinnervate target neurons beyond a site of SCI. This new circuitry can serve as a cellular relay to restore the bidirectional communication between areas above and below the lesion
NSCs can be obtained from different sources including adult NSCs from the forebrain subependymal zone or from the spinal cord [283] and fetal NSCs from fetal brain or spinal cord [209, 272, 274], from embryonic or induced pluripotent stem cells (ESCs, iPSCs) [258, 265, 268], or from somatic cells via fate conversion [264]. However, in comparison to non-CNS cell types, NSCs are more difficult to obtain, and the generation and expansion of cells take several weeks to months. Human adult NSCs obtained during surgery or postmortem [188, 205] and fetal NSCs do not allow for autologous transplantation, and the use of fetal NSCs has some ethical implications. On the other hand, NSCs obtained from pluripotent stem cells or generated by fate conversion can be tumorigenic due to genetic or epigenetic changes [149]. Therefore, caution is advised although NSCs are therapeutically highly promising.
Numerous preclinical studies in SCI have examined the potential of NSCs to mediate functional recovery after SCI. In general, a portion of the transplanted NSCs survive, adopt mature neural phenotypes, and can integrate into the host tissue. However, the mechanisms leading to functional recovery are not always clearly defined. Transplanted cells can provide a substrate for regenerating axons [60, 180, 204], serve as a relay station [26, 123, 168], remyelinate spared projections [32, 54, 55, 111, 138, 174], or provide neuroprotection [72]. More recent studies have also reported that human-induced pluripotent stem cell-derived NSC can promote functional recovery [93, 168, 195, 269].
The first promising stem cell studies in SCI that led to clinical translation were based on the ability to generate large numbers of oligodendrocyte precursors from human embryonic stem cells to remyelinate spared axons and mediate functional recovery [139, 240]. Based on this evidence and additional studies, a phase I study was initiated in 2009 by Geron Corp. to evaluate safety and efficacy of human ES cell transplantation in acute SCI [172]. After five patients underwent the cell transplantation procedure, the clinical trial was discontinued in 2011, apparently for financial reasons [135]. A different company (Asterias Biotherapeutics) has recently relaunched the program in subacute cervical SCI (https://clinicaltrials.gov/ct2/show/NCT02302157).
Two other cell lines that have led to clinical trials in SCI are based on fetal neural stem cells isolated from the brain and spinal cord, respectively. One phase I trial conducted in Canada and Switzerland sponsored by StemCells Inc. (https://clinicaltrials.gov/ct2/show/NCT01321333) in thoracic SCI at least 6 weeks post-injury has recently been completed, and a phase II study in cervical SCI has just started. This study with human fetal brain-derived NSCs is based on recovery mediated by remyelination after rodent SCI [54, 55, 272]. The second phase I study with thoracic AIS A patients sponsored by Neuralstem Inc. is still ongoing (https://clinicaltrials.gov/ct2/show/NCT01772810). The same cells were already used in a clinical trial with amyotrophic lateral sclerosis. Preclinical studies indicated that these cells extend axons in the injured rodent spinal cord for extended distances, form synaptic connections, and form a new relay circuit [168]. Similar results were also obtained with fresh fetal neural stem cells from rats [123, 168], but cells were found to migrate and form ectopic cell masses in CNS regions distant from the lesion site [254], and enhanced locomotor recovery was not observed in a reassessment study [241]. Such potential adverse effects might be due to the means of cell delivery via pressure injection and the lack of functional recovery in a replication study due to poor engraftment.
Conclusions
Progress over the last 30 years has provided important insights into the mechanisms underlying the limited regeneration of the adult nervous system. This has led to a continuously increasing number of clinical trials using pharmacological and cellular approaches. While a major breakthrough in regenerative therapies for spinal cord injury has not yet arrived, several novel concepts and important pathways have been identified and are currently tested in clinical trials. Possibly, single treatments will only be effective in partial injuries and not be able to restore substantial function after severe SCI given the complex interplay between neuron-intrinsic and extrinsic factors contributing to the regenerative failure. However, clinical trials with single treatments will pave the path for future combinatorial approaches to provide meaningful benefits when applied with existing neurorehabilitative and compensatory approaches.
Acknowledgments
This work was supported by the Deutsche Forschungsgemeinschaft (BL414/3-1; SFB 1158), International Foundation for Research in Paraplegia, International Spinal Research Trust and the EU (IRG268282) to A. B., and a Gertrud Reemtsma Foundation predoctoral fellowship to I.S.
References
1.
Aguayo AJ, David S, Bray GM (1981) Influences of the glial environment on the elongation of axons after injury: transplantation studies in adult rodents. J Exp Biol 95:231–240PubMed
2.
Ahmed Z, Bansal D, Tizzard K, Surey S, Esmaeili M, Gonzalez AM, Berry M, Logan A (2014) Decorin blocks scarring and cystic cavitation in acute and induces scar dissolution in chronic spinal cord wounds. Neurobiol Dis 64:163–176PubMed
3.
Aigner L, Arber S, Kapfhammer JP, Laux T, Schneider C, Botteri F, Brenner HR, Caroni P (1995) Overexpression of the neural growth-associated protein GAP-43 induces nerve sprouting in the adult nervous system of transgenic mice. Cell 83:269–278PubMed
4.
Alilain WJ, Horn KP, Hu H, Dick TE, Silver J (2011) Functional regeneration of respiratory pathways after spinal cord injury. Nature 475:196–200PubMedPubMedCentral
5.
Alto LT, Havton LA, Conner JM, Hollis Ii ER, Blesch A, Tuszynski MH (2009) Chemotropic guidance facilitates axonal regeneration and synapse formation after spinal cord injury. Nat Neurosci 12:1106–1113PubMedPubMedCentral
6.
Au E, Richter MW, Vincent AJ, Tetzlaff W, Aebersold R, Sage EH, Roskams AJ (2007) SPARC from olfactory ensheathing cells stimulates Schwann cells to promote neurite outgrowth and enhances spinal cord repair. J Neurosci 27:7208–7221PubMed
7.
Ballermann M, Fouad K (2006) Spontaneous locomotor recovery in spinal cord injured rats is accompanied by anatomical plasticity of reticulospinal fibers. Eur J Neurosci 23:1988–1996PubMed
8.
Bamber NI, Li H, Lu X, Oudega M, Aebischer P, Xu XM (2001) Neurotrophins BDNF and NT-3 promote axonal re-entry into the distal host spinal cord through Schwann cell-seeded mini-channels. Eur J Neurosci 13:257–268PubMed
9.
Barakat DJ, Gaglani SM, Neravetla SR, Sanchez AR, Andrade CM, Pressman Y, Puzis R, Garg MS, Bunge MB, Pearse DD (2005) Survival, integration, and axon growth support of glia transplanted into the chronically contused spinal cord. Cell Transplant 14:225–240PubMed
10.
Bareyre FM, Kerschensteiner M, Raineteau O, Mettenleiter TC, Weinmann O, Schwab ME (2004) The injured spinal cord spontaneously forms a new intraspinal circuit in adult rats. Nat Neurosci 7:269–277PubMed
11.
Barritt AW, Davies M, Marchand F, Hartley R, Grist J, Yip P, McMahon SB, Bradbury EJ (2006) Chondroitinase ABC promotes sprouting of intact and injured spinal systems after spinal cord injury. J Neurosci 26:10856–10867PubMedPubMedCentral
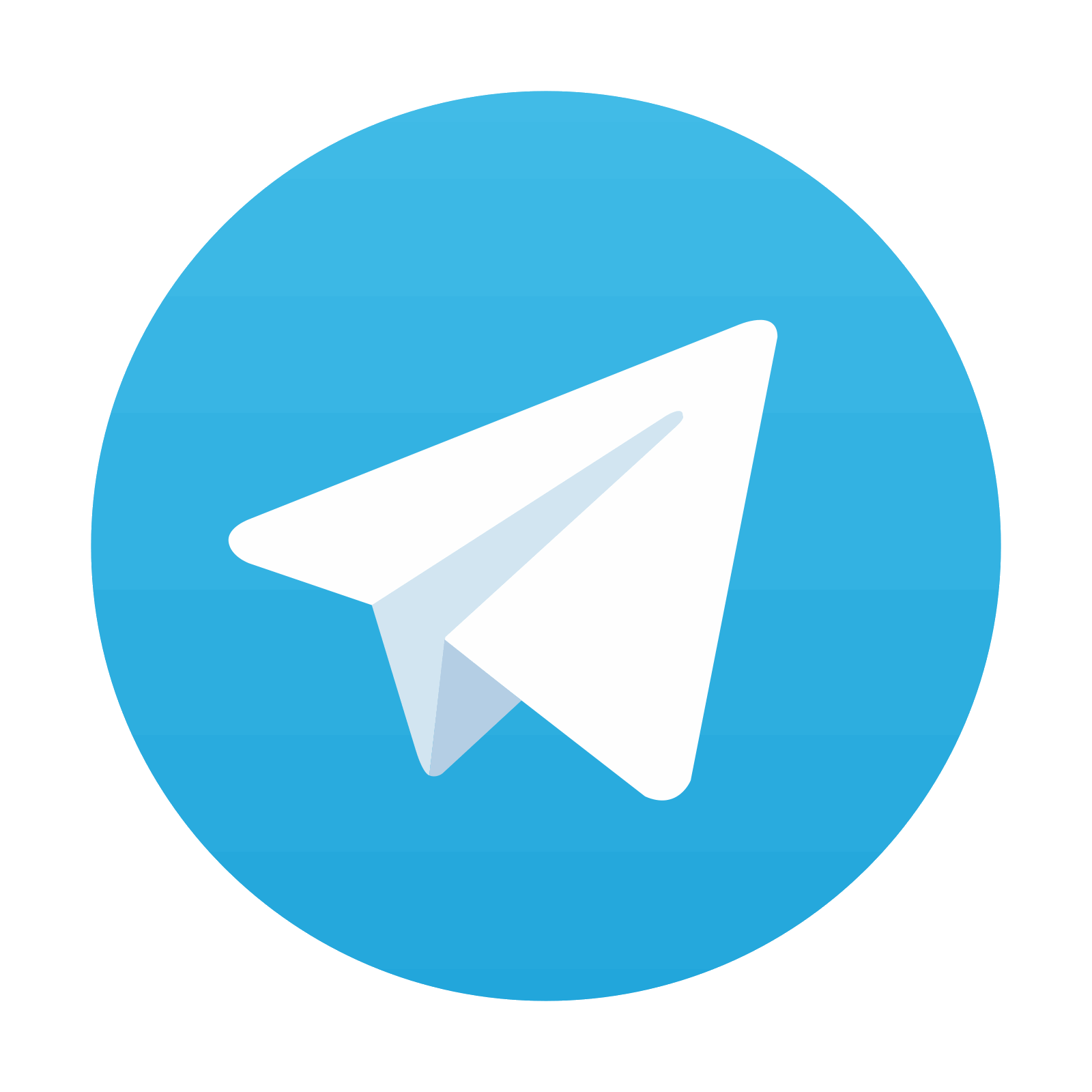
Stay updated, free articles. Join our Telegram channel
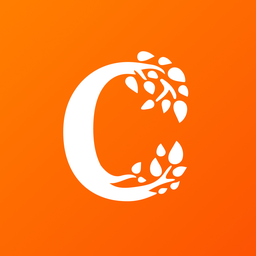
Full access? Get Clinical Tree
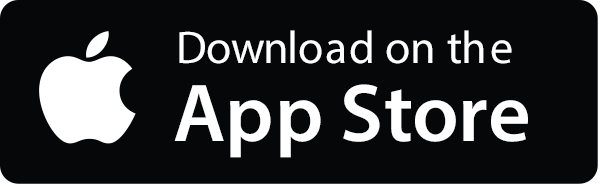
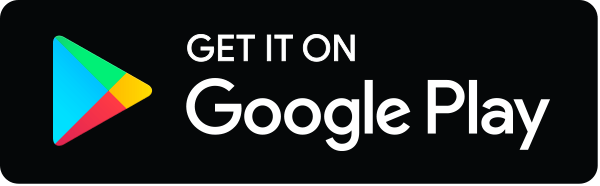