Extensively Coated Femoral Components
William D. Bugbee
C. Anderson Engh
Bone ingrowth, or osseointegration, of cementless, porous-coated femoral stems has proven to be a reliable and successful form of fixation. Clinical experience with proximally and extensively microporous-coated stems has passed two decades and has led to continued enthusiasm for porous-coated stems. The goal of this chapter is to summarize the experience with extensively porous-coated, cylindrical, femoral components. The chapter is divided into six sections covering (a) historical development, (b) stem design features, (c) laboratory investigations of autopsy specimens, (d) surgical principles, (e) surgical technique, and (f) the authors’ long-term clinical experience.
Historical Context
The first femoral implant to demonstrate the possibility of biologic fixation was developed by Austin-Moore in the early 1950s (1). This stem, with large fenestrations, achieved macrointerlock with the skeleton through the placement of bone graft in these fenestrations. At the same time John Charnley was developing the use of acrylic cement for fixation, others, such as Judet et al. (2) and Lord et al. (3), were experimenting with stems with macroporous or rough-textured surfaces. The 1970s was a time of intensive basic experimental research on surface coating and tissue ingrowth. These investigations delineated two basic criteria for bone ingrowth into porous surfaces. First, bone ingrowth into microporous surfaces could be achieved when pore size was between 50 and 500 μm (4). Second, for this bone ingrowth to be achieved, a stable implant with minimal interface motion was necessary. It was evident that the type of porous surface was not nearly as important as having an interface environment that met these two criteria. On the industrial side, technology for the production of microporous surface implants was developed.
In 1977, a US Food and Drug Administration (FDA)-approved clinical trial was initiated in which primary arthroplasties were performed with a fully porous-coated femoral component with a 32-mm head size. In 1982, the results of 120 cases were presented to the FDA, and in 1983, after the FDA approved this implant, modifications were made by the manufacturer: The implant was made in a greater variety of sizes, the porous coating was removed from the distal one-eighth of the stem, and a porous-coated acetabular component was designed. In 1984, the femoral prosthesis was again modified: A Morse taper was applied to the femoral neck to make the femoral head of the prosthesis modular, and the same prosthesis was also manufactured with porous coating applied proximally to less than one-half the length of the stem. For the subsequent 3 years, prostheses with both porous coating levels were used. However, since 1988, our experience has been only with the more extensively porous-coated implant. Throughout this design evolution, patient data have been collected preoperatively and annually postoperatively in a computerized database. An autopsy retrieval program has allowed the study of the type of bone ingrowth into porous-coated implants, their mechanical stability, and the overall bone adaptation that occurs to the implant.
Optimum Design Characteristics of an Extensively Porous-Coated Stem
An extensively porous-coated prosthesis can be defined as one with porous coating on more than 80% of its surface area, with a stem length that allows this porous surface to extend to the narrowest portion of the medullary canal, the femoral isthmus. Most manufacturers provide stems with these characteristics. The Anatomic Medullary Locking (AML) stem (DePuy, Warsaw, IN) was the first stem of this type widely used for primary arthroplasties in the United States. The stem is made of cast cobalt-chrome. The porous surface is a powder-made beaded surface applied through a modified loose sintering process. The beads are of cobalt-chrome alloy, and their size ranges from 187 to 250 μm. The average pore size of the applied coating is 250 μm, with a range of 50 to 400 μm. On the implant itself, the porosity distribution ranges from 87% at the surface of the implant to 23% adjacent to the solid base substrate. The average porosity is 40%. The shear strength at the interface of the porous coating and the solid substrate depends on volume porosity. At 40% porosity, the substrate–coating interfacial shear
strength is 21 MPa. In animal studies, when bone ingrowth occurs, the interfacial strength of the bone–implant has been shown to be as high as 17 MPa for cortical bone and 5 to 6 MPa for dense cancellous bone (5). This 40% porosity has been considered optimal for balancing the strength of the porous coating–substrate interface and that of the porous coating–bone interface. Finite element studies have demonstrated that the sintering process could potentially lessen the fatigue strength of some porous-coated stems and produce stem breakage. This has been demonstrated to be of particular concern with fully porous-coated stems with a core stem diameter of less than 12 mm (6).
strength is 21 MPa. In animal studies, when bone ingrowth occurs, the interfacial strength of the bone–implant has been shown to be as high as 17 MPa for cortical bone and 5 to 6 MPa for dense cancellous bone (5). This 40% porosity has been considered optimal for balancing the strength of the porous coating–substrate interface and that of the porous coating–bone interface. Finite element studies have demonstrated that the sintering process could potentially lessen the fatigue strength of some porous-coated stems and produce stem breakage. This has been demonstrated to be of particular concern with fully porous-coated stems with a core stem diameter of less than 12 mm (6).
A critical design feature of this class of femoral components is its straight, cylindrical, nontapered distal stem geometry. Because the stem is not tapered, the implant does not wedge in place. Fixation depends instead on a so-called scratch fit between the rough external surface of the implant and a similarly shaped bone canal. The bone canal is reamed using intramedullary drills to a diameter slightly smaller in size than that of the stem. Using a straight cylindrical design makes it possible to easily prepare the inside of the femur to match the shape of the stem over a large surface area. The advantage of the extensive coating on a stem of this design is that it makes it possible to achieve biologic fixation through osseointegration over the entire length of the stem. Coating the distal part of the stem is particularly important, because it is the distal part of the stem that most consistently contacts the cortical bone. The cortical bone of the femoral diaphysis also has superior ingrowth characteristics and is of greater strength than cancellous bone. Thus, fully porous-coated stems can be equated to cemented ones in which the surgeon seeks to obtain a complete cement mantle for optimal fixation of the entire stem. It also has been noted that in the few cases where extensively coated stems are used and osseointegration does not occur, fibrous tissue growth into the porous surface usually occurs. This alone, in the majority of cases, is adequate to provide patient satisfaction and radiographic stability. The extensive coating presumably creates a large enough area of interface bonding that fibrous ingrowth is adequate to prevent stem subsidence. The same long-term implant stability has not been observed when bone ingrowth failure occurs with proximally porous-coated stems (7).
Laboratory Investigation of Extensively Coated Stems
Implant retrieval enables understanding of the long-term skeletal response to extensively porous-coated stems. Examining well-functioning stems and the surrounding bone makes it possible to gain valuable insight into the in vivo characteristics of the implant–bone system. In particular, important information has been obtained on mechanical stability, patterns of bone ingrowth, and patterns of bone remodeling.
The shear strength of the bone–porous coating interface has been evaluated in animal studies (4), which have shown that implant bonding occurs as early as 3 weeks after implantation and may reach maximum strength by 8 weeks. The maximum shear strength resulting from bone ingrowth in humans is probably slower.
Based on a determination of the percentage of the porous surface of stem containing bone ingrowth, the maximum interfacial shear strength can be calculated to reach 17 MPa in cortical bone and 5 to 6 MPa in dense cancellous bone (8). These bonds prevent micromotion between the implant and bone, but only at the areas where the two are bonded. Engh et al. (9), performing micromotion studies on autopsy-retrieved femora, showed that when bone ingrowth occurs, the movement between the porous-coated portion of the implant and the surrounding bone is usually less than 20 μm and never exceeds 40 μm. However, with proximally porous-coated implants, the motion between the uncoated and unbonded distal smooth stem is greater. In addition, the amount of this distal (tip) micromotion is related to the extent of the implant surface that is not porous coated. Stems that were 80% coated demonstrated a maximum of 120 μm of motion at the tip, compared with 210 μm of tip micromotion for the 40% proximally coated stems. These data correlate with clinical experience. The incidence and severity of early postoperative thigh pain are greater with proximally porous-coated AML stems than with more extensively coated ones, suggesting that the increased distal-stem tip motion that occurs with the implants with less coating is a source of thigh pain (10).
After micromotion studies were completed, these specimens were embedded in plastic and sectioned for microscopic analysis. Back-scattered electron microscopy was used to study the bone ingrowth producing implant stability (8). In eight specimens studied, the percentage of the porous surface area containing bone ingrowth averaged 35%. In areas where there was bone ingrowth, 67% to 73% of the void space between the pores contained bone. Figure 59.1 illustrates the typical back-scattered electron microscopic appearance of bone ingrowth.
The amount of bone growth within the pores was not influenced by the anatomic location of the ingrowth. However, in concurrence with previous studies that have shown the likelihood of bone ingrowth increasing with proximity of
the porous coating to cortical bone, the largest areas of bone ingrowth occurred in the areas of the femoral diaphysis with the stem fit tightest against the cortices. The greatest amount of bone ingrowth was observed to be consistently near the termination of the porous coating. At this level, compact bone directly adjacent to the porous coating showed haversian systems identical to those in the surrounding cortex.
the porous coating to cortical bone, the largest areas of bone ingrowth occurred in the areas of the femoral diaphysis with the stem fit tightest against the cortices. The greatest amount of bone ingrowth was observed to be consistently near the termination of the porous coating. At this level, compact bone directly adjacent to the porous coating showed haversian systems identical to those in the surrounding cortex.
![]() Figure 59.1. Back-scatter scanning electron micrograph of bone growth into the porous surface of an Anatomic Medullary Locking (AML) implant (15×). |
A different pattern of bone ingrowth was observed in the more proximal areas of these specimens. The ingrowth was less predictable, but it was most frequently observed on the rounded medial and lateral corners of the implant. This bone was cancellous in nature and was usually connected to the outer cortex by hypertrophied trabeculae. This type of connecting bone was usually not visible on clinical radiographs. These patterns of cortical and cancellous bone ingrowth into porous-surfaced extensively porous-coated stems are shown in Figure 59.2. Micromotion studies performed on these same autopsy-retrieved femora before embedding and sectioning showed that although this connecting bone was quantitatively less proximal than distal, it was adequate to stabilize the proximal part of the implant (9).
Autopsy specimens embedded in paraffin were used to microscopically examine the nonbony tissue surrounding some areas of the implant. In the nonossified marrow spaces where micromotion studies had shown minimal motion between the porous implant and the bone, fibrous tissue was frequently observed to grow within the porous surface. In other areas, there was no fibrous tissue reaction, and the marrow appeared normal. In areas where mechanical testing had indicated that the micromotion between the implant exceeded 50 μm, the marrow space was filled with fibrous tissue surrounding the implant. This fibrous tissue was organized differently: It ran parallel to the implant surface. In some cases, this type of fibrous tissue was partially ossified.
In addition, histologic studies have revealed two other findings. First, the ingrowth pattern did not appear related to the in vivo duration of the implant. Second, the relative porosity of the femoral cortex did not affect the amount of bone ingrowth. However, the specimens with the most pronounced osteoporosis tended to have predominantly cancellous or trabecular bone ingrowth rather than compact bone ingrowth (8).
Serial clinical radiographs of a single patient and that patient’s autopsy specimen have been used to study the bone remodeling that occurs as the bone attempts to adapt to the implant (7,11,12,13). Three techniques have been used: subjective evaluation of serial radiographs, computer-assisted videodensitometry of the same clinical radiographs, and dual-energy x-ray absorptiometry (DEXA) analysis of autopsy-retrieved specimens. Analysis of the autopsy-retrieved specimens provides the most accurate quantitative information. There are two problems with subjective evaluation of clinical radiographs: The method is not quantitative, and it is limited by variation in radiographic technique (14). These two deficiencies can be partially corrected by converting these same radiographs to digital images and using a technique termed “histogram-directed equalization” to correct for variations in radiographic technique. A computer program can then be used to quantitate zonal changes on serial radiographs. This method was used to evaluate 15 cementless total hip arthroplasties (15). With an AML prosthesis at 2 years postoperatively, the decrease in density in the proximal periprosthetic bone ranged from 11% to 28%. An increased density was noted adjacent to the stem tip. When serial clinical radiographs were evaluated by this method, no significant progression of periprosthetic resorptive changes was seen for the extensively porous-coated implant between the second and fifth postoperative years, suggesting that some type of equilibrium was achieved at the first 2 years after the arthroplasty.
By far the most sensitive method of determining periprosthetic bone remodeling is through the use of DEXA. By using different absorptions of high- and low-radiation energies, DEXA allows direct measurement of bone mineral content. The femora of 11 patients with well-functioning unilateral hip replacements retrieved at autopsy were analyzed (7). For this study, the contralateral femur was also retrieved and implanted with a matching prosthesis in vitro. This served as the control for bone mineral comparisons: An average decrease in bone mineral content of 23% in the implanted femur (range, 5% to 47%) was observed. Women experienced more bone loss than men. The average bone loss for the remodeled femur was 12% for the men, compared with 31% for the women. Zonal analysis revealed an average decrease in bone mineral content of 42% proximally, 23% in the area adjacent to the midstem, and 6% adjacent to the distal stem.
In these 11 cases, no correlation was observed between the degree of bone loss and the implant diameter of the stem that had been used or between bone loss and the duration of implantation. The most striking finding was the strong inverse correlation between bone mineral content in the control femur and the extent of bone mineral loss in the remodeled
femur (p <0.05; r2 = 0.94). This correlation suggests that the initial bone stock of the femur has the most important influence on the extent of bone remodeling. Patients with low bone mineral content in the proximal femur at the time of surgery appear prone to more bone loss as a result of bone remodeling. Although there is a correlation between the bone mineral content and the mechanical properties of the femur, this finding suggests the intriguing possibility that the biologic state of the bone at the time of implantation has an important influence on the extent of bone remodeling.
femur (p <0.05; r2 = 0.94). This correlation suggests that the initial bone stock of the femur has the most important influence on the extent of bone remodeling. Patients with low bone mineral content in the proximal femur at the time of surgery appear prone to more bone loss as a result of bone remodeling. Although there is a correlation between the bone mineral content and the mechanical properties of the femur, this finding suggests the intriguing possibility that the biologic state of the bone at the time of implantation has an important influence on the extent of bone remodeling.
Bone remodeling continues to be an area of intensive investigation, and the orthopedic community is beginning to understand the influence of the biologic factors, in addition to the mechanical factors, that interact to cause remodeling around cementless implants. The classic explanation of stress bypass by stiff implants does not fully explain such observations. It is increasingly recognized that all implant systems, whether cementless or cemented, elicit similar bone remodeling responses, characterized by loss of bone mineral content in the most proximal regions of the femur (16,17). Extensively coated stems are unique only because they are the most well studied design with respect to bone remodeling.
Clinical correlation of radiographs and autopsy specimens confirms that it is possible to predict bone ingrowth into porous-coated stems based on changes in the appearance of clinical radiographs. The femur appears to respond to an extensively coated implant in a predictable fashion, in that characteristic radiographic patterns can be observed on plain radiographs (18). There are three typical responses: (a) bone ingrowth occurs; (b) bone ingrowth does not occur but the implant is stabilized by fibrous tissue ingrowth; and (c) bone ingrowth does not occur and the stem becomes unstable. Each of these events is recognizable on plain radiographs, and recognition of these patterns is very useful in clinical practice. The typical pattern correlating with bone ingrowth is illustrated in Figure 59.3
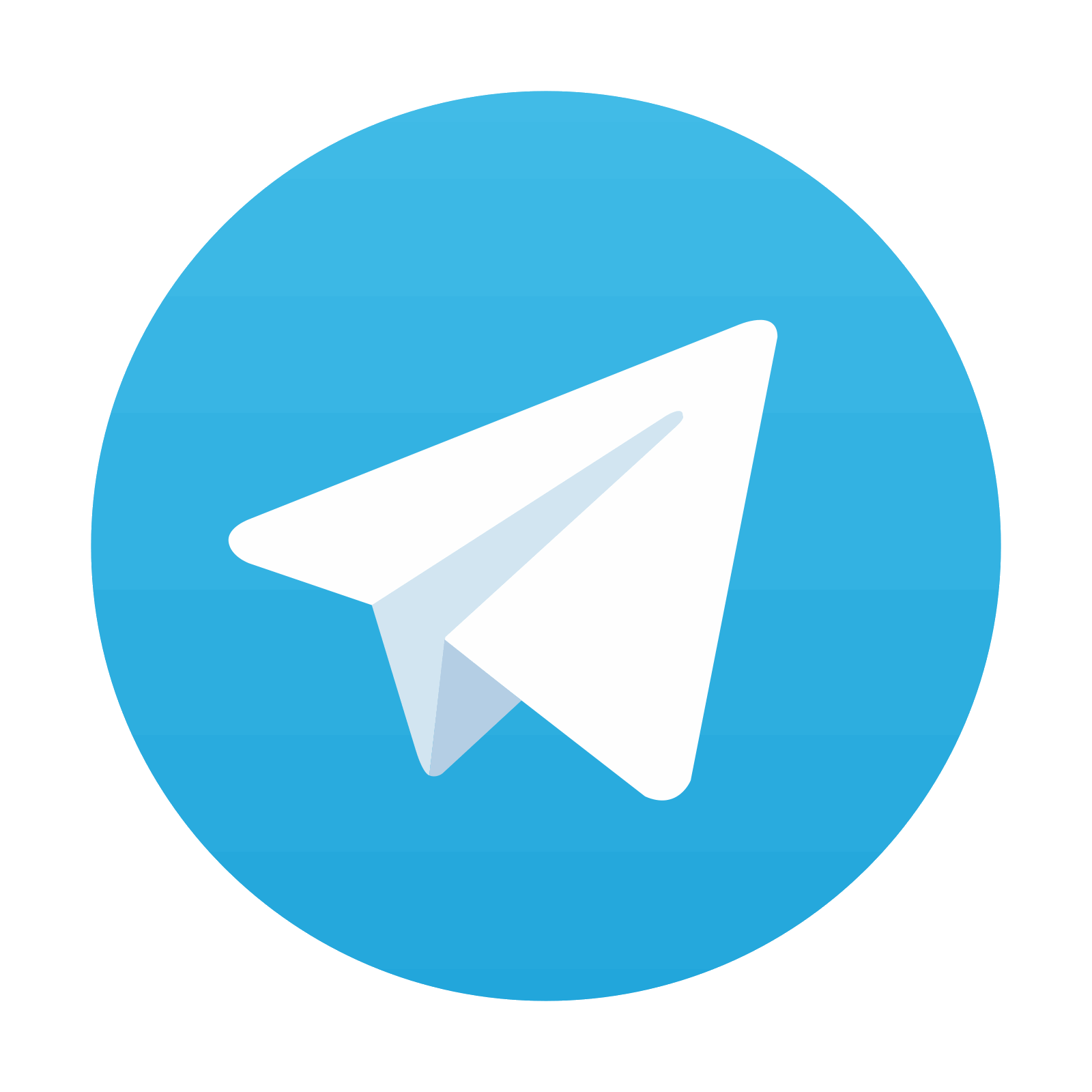
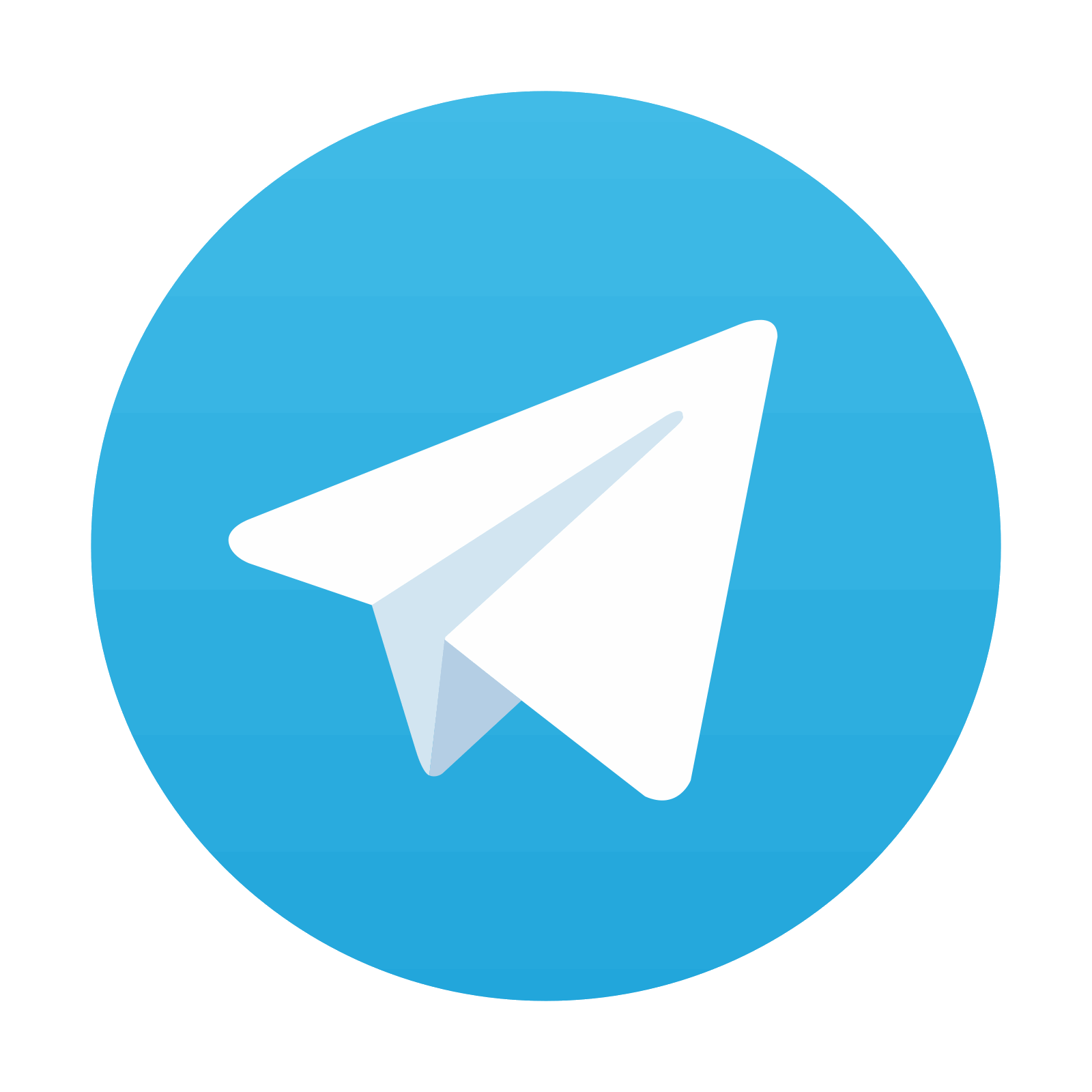
Stay updated, free articles. Join our Telegram channel
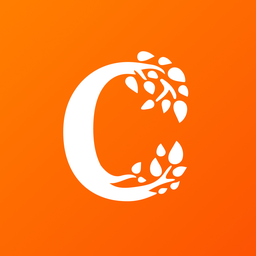
Full access? Get Clinical Tree
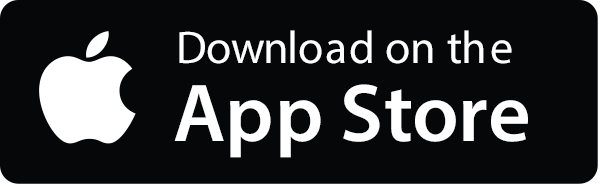
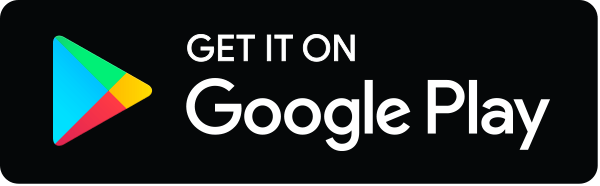