Fig. 4.1
The functional model of bone development based on the mechanostat theory [20] and related approaches [19]. The feedback loop between bone deformation (tissue strain) and bone strength is central to regulation of bone adaptation. Various modulating factors influence aspects of the regulatory system as indicated by the dashed arrows. [Adapted from Rauch F, Schoenau E. The developing bone: slave or master of its cells and molecules? Pediatr Res. 2001 Sep;50(3):309–14. With permission from Nature Publishing Group]
Our understanding of bone adaptation to loading has been greatly enhanced by experimental evidence from animal models [21–27]. Based on this evidence, Charles Turner proposed three fundamental “rules” that predict bone structural adaptations to mechanical stimuli [28]. First, dynamic loading drives bone adaptation (as opposed to static loading). Further, the stimulus for bone adaptation increases with the magnitude or frequency of the loading. Second, only short bouts of mechanical loading are necessary to elicit an osteogenic response. There is a ceiling effect for bone tissue stimulation, whereby bone adaptations are subject to diminishing returns beyond a certain loading frequency or duration. Third, bone cells become accustomed to routine strain and structural change is driven by abnormal strains. These “rules” provide insight into how different intensities and modalities of exercise predict bone adaptation in humans.
During growth, bone can adapt its strength in response to mechanical stimuli through several different mechanisms: (1) periosteal apposition can increase bone cross-sectional area (CSA); (2) periosteal apposition in conjunction with reduced endocortical resorption can increase cortical thickness; and/or (3) modifications to cortical and trabecular microarchitecture (i.e., increased trabecular thickness or number or decreased cortical porosity) can increase tissue density [7, 29]. In contrast, the mature skeleton appears to preferentially adapt to loading through gains in bone density as evidenced by results from animal studies [30]. Further, animal studies also demonstrate a greater capacity of the growing skeleton to adapt to mechanical loading than the mature skeleton [31, 32].
Muscle–Bone Relationship
Frost’s mechanostat theory and the functional model of bone development contend that bone continually adapts to increased mechanical loads through changes in mass, structure, microarchitecture, and strength in order to maintain strains within safe limits [19, 20]. Muscle contractions pose the greatest mechanical challenge to bone (stresses several-fold greater than body weight alone) and are the primary driving force of bone adaptation. If, as suggested by the functional model of bone development, increasing muscle forces provide the stimulus for increases in bone mass, structure, microarchitecture, and strength, it stands to reason that muscle development should precede bone development. Longitudinal data from the University of Saskatchewan Pediatric Bone Mineral Accrual Study (PBMAS) provide evidence for this phenomenon. First, Rauch et al. demonstrated that velocities of total lean body mass (LBM; surrogate of muscle force) precede peak BMC accrual (by DXA) by approximately 6 months in girls and 4 months in boys [33]. Additionally, peak LBM preceded peak BMC in 87 % of girls and 77 % of boys [33]. In a subsequent analysis using hip structural analysis (HSA), velocities of total LBM accrual peaked prior to velocities of bone CSA and estimated bone strength (section modulus) at the narrow neck and femoral shaft by 2–4 months (Fig. 4.2) [34]. These findings support the theory that increases in muscle mass peak prior to increases in bone mass and suggest that the stimulus of muscle drives bone growth. However, the relationship between bone and muscle might not be uniform throughout the skeleton and may be site specific. For example, Xu et al. noted that muscle CSA (surrogate of muscle force) at the tibial shaft (by pQCT) peaked prior to BMC and BMD, but lagged behind that of total and cortical bone CSA [35]. We discuss several studies that imply muscle forces mediate the exercise–bone relationship [36, 37] in greater detail below.
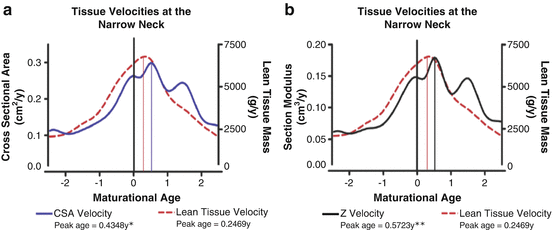
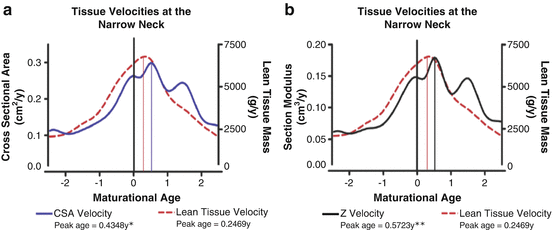
Fig. 4.2
Tissue velocity curves for lean tissue mass, (a) cross-sectional area (CSA) and (b) section modulus at the narrow neck region of the proximal femur aligned by maturation in girls and boys. The maturational age of 0 represents the age at peak height velocity (12.49 years). The solid drop down lines landmark the maturation age at which the peak tissue velocities occur. Single asterisk indicates a significant difference between the age of peak lean tissue velocity (PLTV) and peak CSA velocity. Double asterisk indicates a significant difference between PLTV and peak section modulus velocity. [Reprinted from Jackowski SA, Faulkner RA, Farthing JP, Kontulainen SA, Beck TJ, Baxter-Jones ADG. Peak lean tissue mass accrual precedes changes in bone strength indices at the proximal femur during the pubertal growth spurt. Bone. 2009 Jun;44(6):1186–90. With permission from Elsevier]
How to Measure Bone Adaptation to Exercise
In this section, we briefly discuss commonly used densitometric techniques, but refer the reader to Chap. 5 for more detail regarding the assessment of bone health in the young athlete. DXA is often the imaging device of choice in clinical bone health research due to its relative ease of use, low radiation dose, and ease of assessment of BMC and aBMD at clinically relevant sites such as the lumbar spine and hip. However, DXA’s planar nature is unable to account for bone depth and this limitation precludes it from assessing the distribution of bone mass [38]. As a result, DXA-based measures of bone mass and aBMD are highly influenced by bone size, systematically underestimating aBMD in short individuals and overestimating aBMD in taller individuals [39]. This issue is particularly problematic when assessing bone in the growing skeleton, whether making comparisons between individuals of different sizes or within the same individuals longitudinally. Several strategies have been proposed to account for DXA’s size dependency by adjusting for body size and composition variables such as height, bone area, maturity, and mass [40]; however, even with such adjustments, DXA is still limited by its inability to assess 3D cross-sectional geometry and differentiate between cortical and trabecular compartments.
Bone’s complexity and mechanical competence cannot be adequately described by simply the amount of bone present. As mentioned previously, measures of BMC and aBMD do not reflect where bone is located. As bone bending strength varies exponentially with the distance from the center of mass of the cross section, less material is needed for the same bending strength as the diameter increases. Thus, when investigating bone adaptation to exercise, important properties of whole bone strength such as structure and microarchitecture must be considered. This is highlighted by several animal studies that demonstrated how minimal exercise-induced increases in DXA-derived BMC and aBMD (<10 %) are accompanied by substantial increases (>60 %) in bone strength measured by micro-CT [41, 42]. Further illustrating this phenomenon is a schematic representation that demonstrates marked variation in bone bending strength (section modulus) despite identical aBMD (Fig. 4.3) [43].
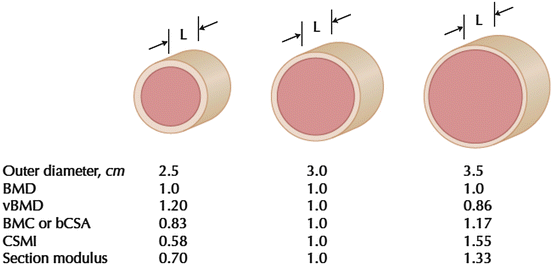
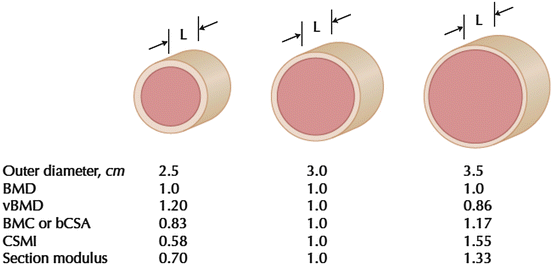
Fig. 4.3
Scale drawing of three-cylindrical cross sections with different outer diameters, but fixed region length (L) and equivalent areal bone mineral density (BMD). The outer diameter, volumetric BMD (vBMD), bone mineral content (BMC), cross-sectional moment of inertia (CSMI), and section modulus are also shown. BMC is not equivalent to CSA excluding spaces occupied by soft tissue (bCSA), but in a cross section they scale linearly. [Reprinted from Beck TJ. Extending DXA beyond bone mineral density: understanding hip structure analysis. Curr Osteoporos Rep. 2007;5:49–55. With permission from Springer Verlag]
HSA is often applied to 2D DXA images to estimate bone cross-sectional geometry and bone strength at three locations of the proximal femur [44]. HSA has figured prominently in the bone literature in the last decade; however, this tool suffers from the same limitations as DXA [45]. Thus, we must consider 3D imaging techniques such as pQCT, HR-pQCT, and MRI to more accurately measure bone structure and microarchitecture, and estimate bone strength.
Unlike DXA, QCT imaging modalities directly measure bone cross-sectional geometry and volumetric BMD (g/cm3) for a given region of interest. pQCT and HR-pQCT are used to assess the appendicular skeleton and are relatively common in pediatric research due to their short scan time and minimal effective radiation exposure. pQCT is typically used to assess bone structure at distal and shaft sites of the tibia and radius. Bone strength in compression can be estimated at distal sites using bone strength index (BSI, mg/mm4; incorporates CSA and BMD) and in bending or torsion at shaft sites using polar strength–strain index (SSIp, mm3; density-weighted section modulus). Additionally, reference data are available for children and young adults for cortical and trabecular BMD, CSA, and cortical thickness [46, 47]. In contrast to pQCT measures at the radius, tibial pQCT measures provide structural information at a weight-bearing site. Despite these advantages, pQCT is limited by a maximum imaging resolution of 0.2 mm and is, therefore, unable to accurately assess trabecular bone microarchitecture, or separate cortical and trabecular bone in regions where the cortex is quite thin, such as the distal radius [48]. HR-pQCT, on the other hand, has an imaging resolution of 82 μm. This resolution permits accurate assessment of trabecular microarchitecture, such as trabecular number and thickness [49]. Cortical porosity can also be quantified using customized software [50, 51] and compressive bone strength can be estimated using finite element analysis [52]. Although limited to assessments of the peripheral skeleton, strong correlations are observed in adults between bone stiffness measures at distal sites by HR-pQCT and finite element analysis and at the lumbar spine and proximal femur using central QCT [53]. Thus, the mechanical competence of sites such as the distal radius and distal tibia are likely reflective of central, clinically relevant sites. Nevertheless, making comparisons between studies can be challenging since pQCT and HR-pQCT acquisition and analysis protocols are not yet standardized.
MRI is a unique bone-imaging tool as it does not emit ionizing radiation and can acquire multiplanar images without repositioning [54]. MRI is also able to assess trabecular and cortical bone using recent image acquisition and processing techniques [55]. Unlike pQCT, MRI is able to scan whole bones and several limbs simultaneously. However, MRI technology is expensive and scan times are considerably longer than DXA and pQCT, ranging from 10 to 30 min depending on the imaging sequence used [56]. Further, quantification of trabecular microarchitecture measures such as thickness and number are challenging and techniques are still being developed. Thus, until recently, the pediatric MRI literature has focused on bone structure as opposed to bone microarchitecture [57, 58].
Advances in bone imaging technologies now permit us to evaluate the subtle adaptations in bone structure and microarchitecture that underpin changes in bone strength across the lifespan. In addition, these technologies are expanding our understanding of how the skeleton adapts to exercise beyond what is already known from traditional DXA-based techniques.
How Exercise Influences Bone Development in Children and Adolescents
We have known for more than two decades that lifestyle habits adopted during childhood and adolescence have the potential to prevent osteoporosis [59]. The positive influence of exercise on bone development is summarized in several excellent reviews [5–12]. Specifically, there is strong evidence to suggest that pre- and early puberty may provide a “window of opportunity” during which the skeleton is particularly responsive to loads associated with weight-bearing activity [9, 15]. In contrast, we know less about the mechanisms underpinning bone’s adaptation to exercise in later adolescence [5, 60–63]. This may be due, in part, to the reliance on DXA in previous studies of this age group, which may have prevented subtle, but important adaptations in bone structure from being captured.
Before discussing details of important studies in this area, we briefly review the normal pattern of bone accrual in childhood and adolescence. Several longitudinal studies have advanced our understanding regarding the timing and magnitude of bone mineral accrual during the growing years [35, 64–66]. One of the most widely cited studies, the University of Saskatchewan PBMAS, followed approximately 200 healthy children for 7 years and conducted annual bone mass measures by DXA [64]. The authors controlled for maturational differences by aligning children on a common maturational landmark, peak height velocity (PHV), and found that total body bone mineral accrual occurred about 1.4 years earlier in girls, and was of a smaller magnitude than in boys. Approximately 35 % of total body and lumbar spine BMC and greater than 27 % of femoral neck BMC was accrued during the 2 years around PHV [4]. Further, 33–46 % of adult BMC was accrued across the entire 5-year period of adolescent growth [65]. This represents double the bone mass that will eventually be lost between the age of 50 and 80 years in women [67]. This period is also critical for development of bone structural properties that contribute to bone strength [35, 66] Thus, it is during childhood and adolescence that the foundation for adult bone health is established. While our skeletal blueprint is largely determined by genetics [68–70], we know that exercise and physical activity play a key role in optimizing bone accrual during growth.
Intervention Studies
Targeted bone-loading programs have traditionally been implemented in elementary schools due to the ease with which large numbers of children from diverse backgrounds can be reached. Effective programs incorporated dynamic, high-impact activities that were of short duration, elicited “unusual” strains and were separated by rest periods, thus mirroring the principles derived from the animal literature [71]. These studies ranged in duration from 3 to 48 months, and most used DXA to monitor exercise-related gains in bone mass [5, 12]. Importantly, children assigned to exercise intervention groups gained significantly more bone mass (1–6 %) at the spine and hip compared with children in control groups [5].
In the longest school-based randomized controlled trial conducted to date, the University of British Columbia’s Healthy Bones Study (HBS), children (aged 9–11 years) attending schools randomized to the exercise group participated in 10–12 min of high-impact jumping activities, three times/week [72, 73]. After the first school year, girls and boys attending intervention schools demonstrated significantly greater gains in bone mass at the femoral neck and lumbar spine compared with children attending control schools; however, in girls the intervention effect was only apparent in those who were early pubertal (Tanner stage 2 or 3) at baseline [74, 75]. After 2 school years, significant gains in femoral neck (5 %) and lumbar spine (4 %) BMC were observed in girls [72] and gains in femoral neck (4 %) BMC were observed in boys [73]. The HBS, and others like it, highlights that a simple exercise program, which requires very little time in the school day, may enhance children’s bone health.
Animal models clearly demonstrate that the skeleton adapts to mechanical loading by adding bone to the periosteal surface of long bone shaft sites where strains are the greatest [71, 76]. Although small in magnitude, these subtle structural adaptations confer dramatic increases in experimentally measured bone strength [41, 42]. As mentioned previously, DXA is unable to capture such adaptations to exercise. Only 11 (8 involved only girls) of the intervention trials conducted in the last decade used imaging tools such as HSA, pQCT, or MRI to assess exercise-induced changes in bone structure and strength [12]. In the HBS trial, the greater gain in femoral neck BMC in early pubertal girls in the intervention group was associated with a 4 % greater increase in femoral neck bone strength (section modulus, HSA) compared with controls after 7 months [29]. This strength gain was attributed to reduced endosteal resorption, leading to a greater increase in CSA and a thicker cortex in the intervention group. In contrast, intervention-related gains in femoral neck bone strength were only observed in boys after the second year of the trial [73]. The apparent sex difference in the timing of structural adaptations to the HBS intervention is likely related to maturity status. At baseline, 60 % of girls were early pubertal, whereas the majority of boys were prepubertal. The later adaptation in bone strength in boys may be a result of advanced maturity (77 % advanced to early- or peri-puberty) over the second year of the study and/or the prolonged intervention. These findings suggest that early puberty may be a window of opportunity for femoral neck bone strength adaptations. A more intense exercise intervention may also be necessary to confer structural adaptations at the hip during prepuberty.
The influence of maturity status on bone structural adaptations to exercise may also vary with skeletal site. In the Action Schools! BC (AS!BC) trial, which involved short bouts of classroom-based exercise (including ~3 min/day of jumping), girls in intervention schools that reported high compliance (≥80 %) demonstrated 5 % greater gains in femoral neck bone strength (section modulus, HSA) compared with girls in control schools [77]. Conversely, an intervention effect was not observed at either the distal or shaft site of the tibia (by pQCT) in girls [78], the majority of whom were early pubertal at baseline. As prepubertal boys in the intervention group demonstrated a 4 % greater gain in distal tibia bone strength (BSI) compared with controls, it is possible that the appendicular skeleton is more responsive to loading during prepuberty. Interestingly, prepubertal girls who completed 7 months of a drop-jumping program (three times/week) did not demonstrate greater gains in MRI-derived bone strength at the mid-femur compared with girls in the control group [79]. As the jumps were unidirectional, more dynamic loads may have been necessary to elicit an osteogenic response.
Whereas the aforementioned trials all included a specific bone-loading component, the Malmö Pediatric Osteoporosis Prevention (POP) study investigated whether increasing curriculum time for physical education could enhance bone accrual. In this 5-year controlled trial, children in the intervention school (aged 6–9 years at baseline) participated in 40 min of daily physical education (200 min/week) compared with the usual 60 min/week of physical education in control schools. After the first 2 years of the intervention, no differences in bone structure (using HSA) were observed between intervention and control girls [80, 81]; however, girls in the intervention group gained significantly more lumbar spine and total body BMC and aBMD than control girls [82]. Over the 5-year study period, intervention girls gained significantly more femoral neck BMC and spine aBMD (by DXA), and had 8 % greater cortical area at the tibial shaft and 9 % greater total area at the radial shaft (by pQCT) compared with girls in the control group [83]. Interestingly, the associated 8–13 % greater estimated bone bending strength (SSIp) at both shaft sites in the intervention group was not significantly different from girls in the control group [83]. Although more robust sample sizes are necessary to confirm these findings, this study suggests that a general exercise program without a specific bone-loading component may enhance bone health in young girls.
School-based intervention trials are challenging to implement and their success depends largely upon participant and teacher compliance. Within the current literature, significant heterogeneity exists across school-based intervention trials regarding the type, intensity, frequency, and duration of the interventions, as well as the imaging tools, scan acquisition, and analysis procedures [12]. Nevertheless, the response to skeletal loading appears to be sex- and maturity specific. Convincing evidence supports the role of high-impact exercise in augmenting skeletal development in pre- and early pubertal girls. Further work is needed to better understand the structural and microarchitectural adaptations to the loads associated with weight-bearing activity including the optimal dose necessary to elicit meaningful benefits.
Observational Studies
Observational studies, including those of groups of athletes subjected to different loading conditions and of habitual physical activity, represent the largest body of evidence to support the positive association between physical activity and bone health during growth. These studies have traditionally relied on DXA technology, and have shown significant bone mass benefits in children who participate in weight-bearing sports such as gymnastics, tennis, running, and ballet compared with nonathlete groups [84–87]. Similarly, leisure-time physical activity is a significant predictor of bone mass accrual in girls and boys [64, 88]. With increased use of 3D imaging tools, we are now gaining insight into the bone structural advantages that are associated with weight-bearing activity. Thus, in this section we focus on observational studies that employed such tools.
Racquet Sports
Athletes in racquet sports such as tennis and badminton provide a unique model for investigating bone adaptation to loading, as within-subject comparisons of the playing and non-playing arms allows control of confounding factors such as genetics, hormones, and diet. The seminal cross-sectional DXA study by Kannus and colleagues [84] paved the way for investigations using more sophisticated imaging tools. Side-to-side differences in BMC were compared in the playing vs. non-playing arms of female racquet sport players and controls. Not surprisingly, athletes had significantly greater side-to-side differences compared with controls (3–5 % vs. 9–16 %). However, more interestingly, players who initiated training prior to menarche had side-to-side differences nearly twice that of players who began training after menarche, suggesting that the skeletal benefits of weight-bearing activity are maximized during the premenarcheal years [84].
The bone mass advantage in the playing arm of female racquet sport athletes is also associated with significant bone strength benefits. For example, bone strength (polar second moment of area) measured with MRI at distal and shaft sites of the humerus in young female tennis players was 11–23 % greater in the playing arm than in the non-playing arm [89]. Similarly, side-to-side differences in pQCT-derived BMC, bone CSA, and strength (BSI) at distal and shaft sites of the radius and humerus were significantly greater in female racquet sport athletes compared with controls. As in the Kannus et al. study, the side-to-side differences in bone structure and strength were double the magnitude in women who began racquet sport training prior to menarche compared with women who began training after menarche (Fig. 4.4) [90]. Finally, in the only prospective study of racquet sport athletes conducted to date, 12-month changes in MRI-derived bone geometry (total and cortical area) were significantly greater among pre- and peri-pubertal female competitive tennis players compared with postpubertal players [91]. Collectively, these findings support the existence of a “window of opportunity” during pre- and early puberty where the skeleton is particularly sensitive to mechanical stimuli.
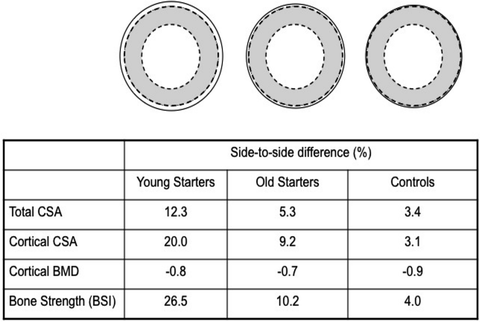
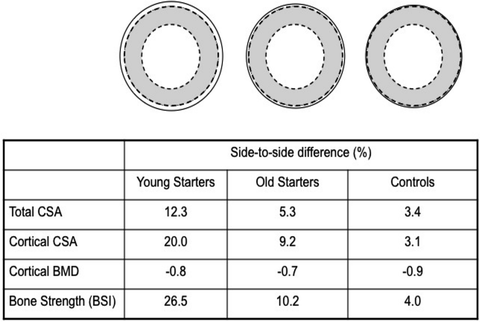
Fig. 4.4
Average side-to-side differences in humeral midshaft total bone CSA, cortical CSA, cortical bone mineral density (BMD), and bone strength index (BSI) between the playing and non-playing arm in female racquet sport athletes as measured with peripheral QCT. The solid line represents the playing arm (or dominant arm in controls). [Adapted from Macdonald HM, Ashe MC, McKay HA. The link between physical activity and bone strength across the lifespan. Int J Clin Rheumatol; 2009 Aug;4(4):437–63. With permission from Future Medicine, Ltd.]
These racquet sport studies also suggested that gains in bone structure at shaft sites and distal sites (total and cortical area) during pre- and early puberty were attributed to bone accrual on the outer bone surface (periosteal expansion) as opposed to endosteal apposition [89–91]. In contrast, training initiated after puberty was associated with greater bone apposition on the endosteal surface, conferring little benefit to bone bending strength [89]. These maturational differences in bone adaptation are likely a result of rising estrogen levels throughout puberty, which inhibit resorption on the endosteal surface during rapid growth and promote bone formation in later puberty [92].
Gymnastics
Artistic gymnastics incurs an extremely high mechanical stimulus to the skeleton (ground reaction forces greater than ten times body weight [93]) and thus, provides a unique model for examining the effects of loading on bone. Gymnasts consistently demonstrate greater bone density, structure, and strength in the upper and lower limbs compared with their non-gymnast peers, and a number of studies in recent years have documented these skeletal advantages using pQCT [94–98].
As with racquet sport athletes, substantial adaptation on bone surfaces (estimated by changes in CSA) at shaft sites contributes to greater bone strength in gymnasts. In a cross-sectional study of 5–11 year old prepubertal boys and girls, the 5–7 % greater total and cortical CSA at the radial shaft of elite gymnasts contributed to 9 % greater estimated bone strength (SSIp) compared with non-gymnast controls [95]. Similar findings were recently reported in 6–11 year old non-elite gymnasts (<16 h/week gymnastics) (Fig. 4.5) [96] and in 4–9 year old recreational current and ex-gymnasts (at least 45 min/week of gymnastics) [97]. Distal sites, on the other hand, demonstrate greater bone strength through adaptations to bone density. Recreational gymnasts and ex-gymnasts demonstrated 6–8 % greater total BMD at the distal radius, which contributed to 22–25 % greater estimated bone strength (BSI) [97]. Collectively, these findings suggest that even recreational gymnastics can provide skeletal benefits in prepuberty.
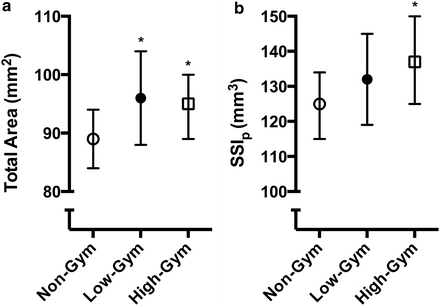
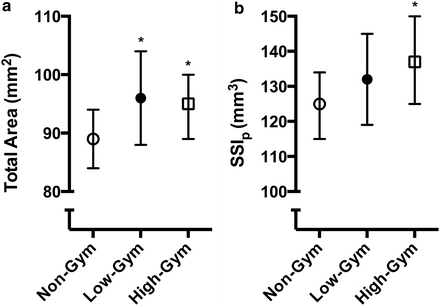
Fig. 4.5
(a) Bone structure (total area) and (b) estimated bone strength (strength–strain index, SSIp) at the proximal radius (66 % site) measured with peripheral quantitative computed tomography (pQCT) in prepubertal female non-gymnasts (Non-Gym), low-training volume gymnasts (Low-Gym), and high-training volume gymnasts (High-Gym). Asterisk indicates significantly different from Non-Gym. Bars represent 95 % confidence intervals (Based on data from [96])
Benefits to bone structure and strength associated with gymnastics training appear to persist into late adolescence. A small cross-sectional study examined bone strength and structure (by pQCT) in post-menarcheal girls (n = 16, mean age 16.7 years) who were ex-gymnasts but had participated in gymnastics (>5 h/week for at least 2 years) throughout early puberty. Ex-gymnasts had 19 % greater trabecular BMD and 25–26 % greater cortical and total CSA at the distal radius, which together conferred 34 % greater estimated bone strength (BSI) compared with non-gymnasts. Similarly, total and cortical CSA were 22–33 % greater in ex-gymnasts at the radial shaft, and the larger bone area was associated with 46 % greater bone strength (SSIp) compared with non-gymnasts [98].
While prospective studies investigating the role of gymnastics on bone accrual are limited [85, 99], the Young Recreational Gymnast Study recently demonstrated that recreational gymnasts between 4 and 9 years of age at baseline had 3 % greater total body and 7 % greater femoral neck BMC over 4 years of follow-up compared with their non-gymnast peers [85]. Subsequent analyses in the same cohort using HSA demonstrated that recreational gymnasts also have 3–6 % greater CSA at all three sites of the femoral neck and 6–7 % greater estimated bone strength (section modulus) at the narrow neck and intertrochanter compared with non-gymnasts [94]. Conversely, ex-gymnasts, the majority of whom ceased participation between the first and second measurement, did not demonstrate any advantages in bone parameters compared with non-gymnasts [85, 94]. In summary, participation in gymnastics, whether elite or recreational, is associated with significant bone health benefits in young girls. However, maintenance of the benefits associated with recreational gymnastics appears to be dependent on continued participation.
Leisure-Time Physical Activity
As not all children are engaged in activities such as gymnastics or racquet sports, we must also consider the influence of more general, leisure-time physical activity on bone health. Current physical activity guidelines recommend that children and adolescents engage in 60 min/day of moderate to vigorous physical activity to achieve health benefits [100, 101]. Guidelines also recommend that children and adolescents engage in muscle and bone-strengthening activities three times/week [100, 101]. While the specific physical activity prescription for optimal bone health is still unknown, the important role of weight-bearing physical activity for shaping skeletal structure throughout the lifespan is well documented.
Similar to the variation in bone imaging procedures discussed previously, there is considerable variation across observational studies in the assessment of physical activity. Measurement techniques range from subjective self-report questionnaires to objective monitoring devices such as accelerometers. Questionnaires are often the tool of choice because they are cost-effective, easy to administer, and are a low burden to the participant [102]. However, self-report questionnaires are subject to recall bias, and while they can provide contextual information regarding physical activity (setting and type of physical activity), questionnaires do not adequately capture physical activity intensity. In contrast, accelerometers can measure physical activity intensity, frequency, and duration. Although accelerometers were originally designed to estimate energy expenditure and not ground reaction forces, strong correlations between ground reaction forces and both accelerometer counts and raw acceleration output suggest they are an appropriate tool for estimating mechanical loads associated with weight-bearing activity [103].
Regardless of physical activity measurement technique, observational studies, both cross-sectional and longitudinal, consistently demonstrate that more active children and adolescents have enhanced bone health and accrue more bone mass and strength compared with their less active peers [36, 37, 64]. For example, vigorous2 physical activity (derived using accelerometry) was a significant predictor of estimated femoral neck bending and compressive strength (by HSA) in pre- [104, 105] and early pubertal girls [105]. Similarly, 9–13 year old girls in the highest physical activity quintile (via self-report questionnaire) demonstrated 8–9 % greater estimated bone strength and 3–4 % greater outer bone circumference (periosteal circumference) at the tibial shaft and metaphysis (by pQCT) compared with their peers in the lowest physical activity quintile [106]. Finally, prepubertal girls who reported participation in high-impact activity (by questionnaire) demonstrated 7 % greater estimated bone strength (polar cross-sectional moment of inertia, CSMI) and 6 % greater cortical thickness at the tibial shaft (by pQCT) compared with girls who engaged in low-impact activities [107]. While these cross-sectional studies clearly highlight a strong association between weight-bearing activity and bone strength in prepubertal girls, the relationship is less clear in adolescent girls. Two pQCT studies reported no significant associations between self-reported weight-bearing activity or overall physical activity and estimates of bone strength at the tibia and radius [108, 109], while the only HR-pQCT study to date demonstrated a significant relationship between impact physical activity (by questionnaire) and total BMD, trabecular BMD, and trabecular number at the distal tibia in 15–20 year old girls [110]. There is a need for prospective studies to examine the structural and microarchitectural adaptations associated with physical activity in adolescence.
Whereas the aforementioned cross-sectional studies provide only a snapshot of the physical activity–bone relationship, prospective studies such as the University of Saskatchewan PBMAS allow us to more closely examine the influence of physical activity on normal bone accrual, while controlling for maturation using age at PHV. In their 7-year study, Bailey and colleagues demonstrated that girls in the highest quartile of physical activity (via self-report questionnaire) gained 11–18 % more BMC at the femoral neck, lumbar spine, and total body compared with girls in the lower quartile. In a subsequent analysis of the PBMAS cohort, self-reported physical activity was also a significant predictor of bone CSA and estimated bone strength (section modulus, HSA) at the femoral neck [36, 64]. Interestingly, physical activity was no longer a significant predictor of bone CSA and section modulus once lean mass (surrogate of muscle force) was entered into the multilevel model, suggesting that muscle forces mediate the relationship between bone structure and physical activity [36]. Similar findings were demonstrated in the Iowa Bone Development Study, an ongoing longitudinal study of bone health during childhood, adolescence, and young adulthood. Moderate to vigorous physical activity, assessed by accelerometry, positively predicted bone CSA and strength (section modulus, HSA) from age 5 to 11 [37]. As in the PBMAS, physical activity was no longer a significant predictor of bone outcomes when lean mass was entered into the multilevel model, again suggesting that muscle forces mediate the association between physical activity and bone health. Moving forward, there is a need for longitudinal studies that use HR-pQCT, pQCT, or MRI to examine the influence of habitual physical activity on bone structure and strength.
Triad Populations
Recent reviews highlight that skeletal benefits of weight-bearing exercise may not be conferred to adolescent athletes with menstrual dysfunction [111, 112]. Specifically, athletes engaged in repetitive loading activities such as running and ballet who experience menstrual disturbances often have low aBMD at non-weight-bearing sites (lumbar spine and distal forearm) [113, 114]. Further, bone health in amenorrheic athletes may not only be compromised compared with eumenorrheic athletes, but may also be lower than that of sedentary women [115]. This was illustrated in a DXA-based study of 12–18 year old female endurance athletes and controls in which amenorrheic athletes had 7–10 % lower aBMD at the spine and whole body compared with their eumenorrheic peers and healthy controls [113]. Despite similar LBM across groups, amenorrheic athletes also had 12 % lower aBMD at the hip compared with athletes without menstrual dysfunction [113].
While studies evaluating “catch-up” of bone accrual during adolescence are sparse, Barrack and colleagues followed adolescent endurance runners for 3 years and showed that despite weight gain and greater number of menses per year, 13 of 15 runners with low bone mass at baseline (1 or 2 SD below age- and sex-specific reference data) exhibited low bone mass at follow-up [116]. Nevertheless, two athletes in this cohort demonstrated significant increases in lumbar spine aBMD to within a normal range over the 3-year follow-up [116]. Additional prospective studies are required to evaluate the potential for catch-up during adolescence. Given that the greatest accrual of bone mass occurs in the 2 years around PHV (approximately age 11–13 in girls) [64], decreased bone accrual during adolescence is of great concern for immediate bone fragility and future skeletal health.
DXA-based studies have helped to shed light on bone health issues in athletic populations considered at risk for developing the female athlete triad. As a result, the American College of Sports Medicine now recommends DXA scans in females with oligomenorrhea or amenorrhea, disordered eating or a stress fracture [117]. However, clinicians must keep DXA’s limitations in mind when interpreting scans, including the systematic underestimation of aBMD in smaller individuals and the challenges presented by changes in body size and composition.
Recently, disparities between amenorrheic and eumenorrheic athletes have also been uncovered using 3D techniques. Ackerman and colleagues [114, 115] used HR-pQCT to assess bone structure, microarchitecture, and strength in amenorrheic and eumenorrheic endurance athletes and healthy controls aged 15–21 years. While bone structure (bone area at the distal radius and tibia) was similar in both groups of athletes, regardless of menstrual dysfunction status, amenorrheic athletes had lower trabecular number and greater trabecular separation at the distal tibia and lower trabecular density at the radius compared with eumenorrheic peers and healthy controls [114]. Subsequent analyses using finite element analysis identified lower estimated bone strength (failure load and stiffness) in amenorrheic athletes compared with nonathletic controls at the distal radius. Moreover, while eumenorrheic athletes demonstrated a significant bone strength advantage at the weight-bearing tibia compared with nonathletic controls, amenorrheic athletes reaped no such benefits (Fig. 4.6) [115]. These studies suggest that amenorrheic athletes are at a significant skeletal disadvantage compared with their normally menstruating peers. Additionally, weight-bearing exercise may not be able to compensate for the associated detrimental effects of estrogen deficiency. This field of study would benefit from prospective, longitudinal studies of bone health in those with menstrual dysfunction.
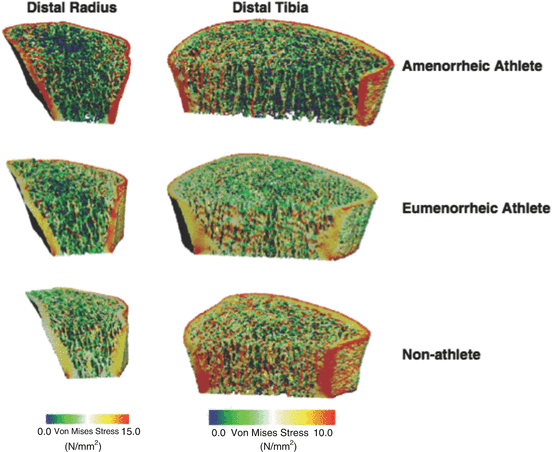
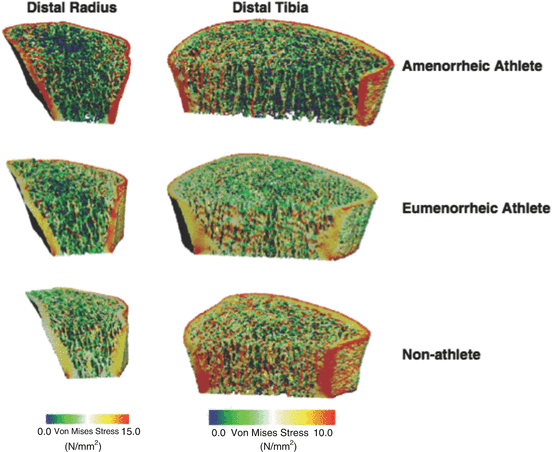
Fig. 4.6
Three-dimensional rendering of distribution of von Mises stresses at the distal radius (left) and tibia (right) using high-resolution peripheral quantitative computed tomography (HR-pQCT) and finite element analysis in an amenorrheic athlete, eumenorrheic athlete, and nonathletic control. Each bone was subjected to a uniform axial compression of 1,000 N for the distal radius and 1,000 N for the distal tibia. The colors indicate the stress levels, with red indicating high stresses and blue representing low stresses (Reprinted from Ackerman KE, Putman M, Guereca G, Taylor AP, Pierce L, Herzog DB, et al. Cortical microstructure and estimated bone strength in young amenorrheic athletes, eumenorrheic athletes and non-athletes. Bone. 2012 Oct;51(4):680–7. With permission from Elsevier)
Do the Benefits Achieved During Growth Persist into Adulthood?
It is currently unknown whether exercise-related gains in bone structure and strength are maintained into adulthood and associated with reduced fracture risk later in life. Results of elegantly designed animal models support lifelong benefits of exercise during growth on bone structure, strength, and fracture resistance [118]. A similar prospective study has yet to be conducted in humans due to obvious methodological challenges. However, we know that exercise and physical activity engagement during childhood and adolescence predict young adult bone health, as demonstrated in observational [119, 120] and athletic studies [121, 122]. Specifically, results from two key prospective studies, the Penn State Young Women’s Health Study (YWHS) and the University of Saskatchewan PBMAS, indicate that girls who were most active during adolescence maintained bone mass and strength advantages over their less active peers in later adolescence and young adulthood. In the YWHS, premenarcheal girls in the most active tertile at baseline (self-report questionnaire; mean age 11.9 years) had 10–11 % greater estimated femoral neck strength (HSA) at age 17 compared with the less physically active girls [119]. Similarly, in a follow-up study of participants in the PBMAS cohort young women and men 23–30 years of age who were most active in adolescence remained more active in adulthood than their peers. Further, those women who were in the upper quartile for physical activity during adolescence had 9–10 % greater adjusted total hip and femoral neck BMC, 10–12 % greater adjusted cortical bone area and content at the tibia diaphysis and 3 % greater adjusted trabecular content at the distal tibia in adulthood compared with their inactive peers [120, 123].
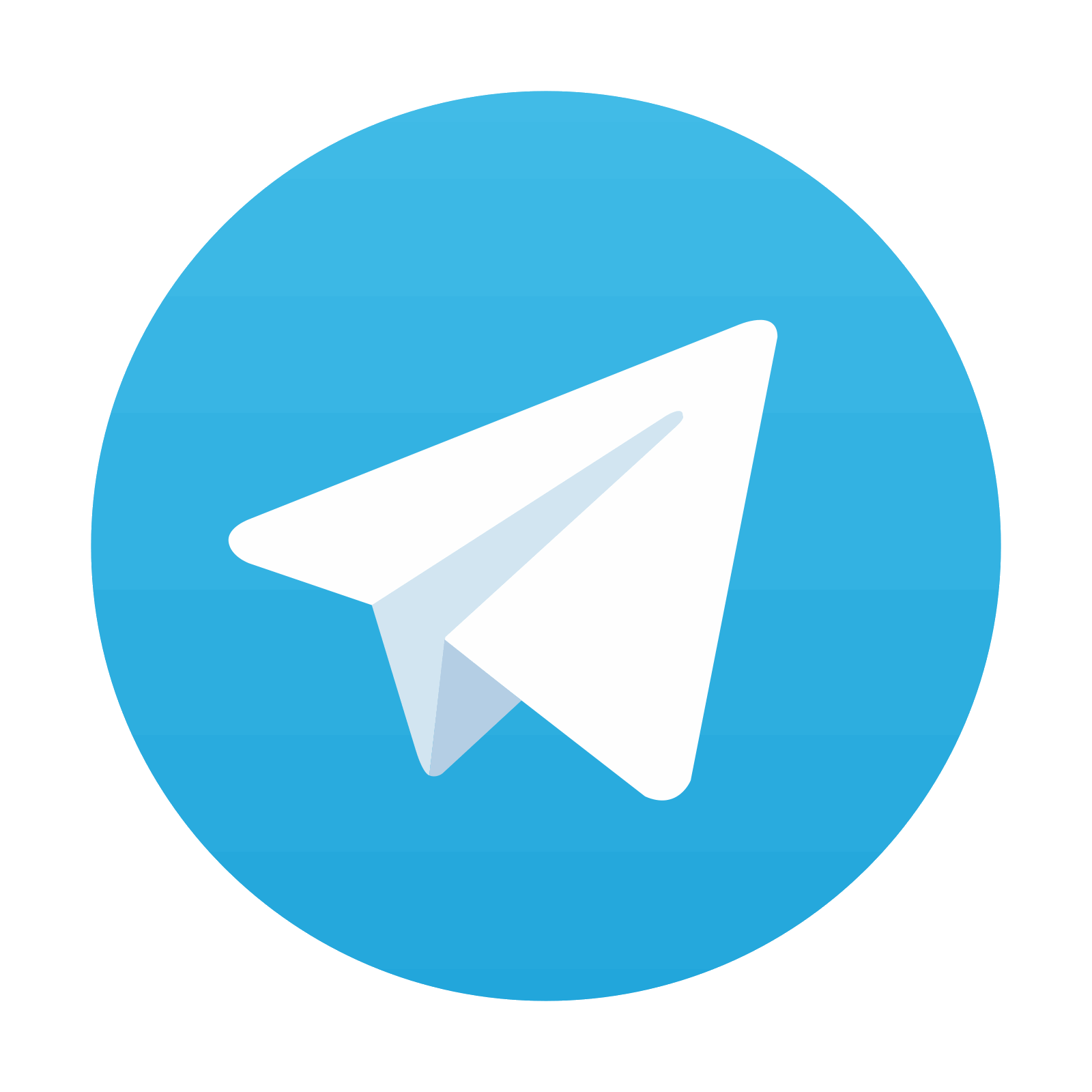
Stay updated, free articles. Join our Telegram channel
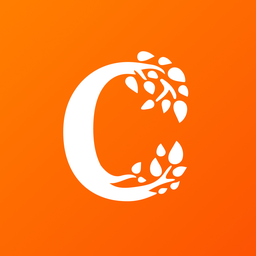
Full access? Get Clinical Tree
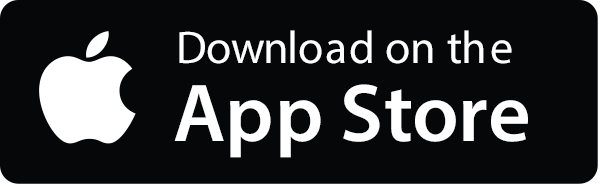
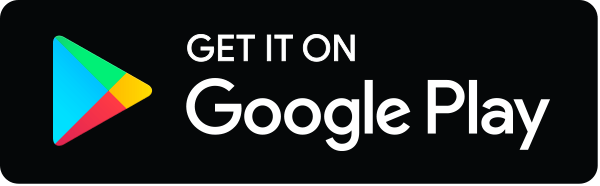