Articular cartilage is a uniquely ordered tissue that is designed to resist compression and redistribute load, but is poorly equipped for self-repair. The chondrocyte is the only resident cell type, responsible for maintaining a specialised and extensive matrix that is avascular and lacks innervation. These attributes, as well as the slow turnover rate of aggrecan and type II collagen in mature articular cartilage, present a considerable challenge to the tissue engineer. Similarly, those attempting to halt the progression of cartilage erosion must contend with these unusual characteristics. This review explores the gaps in our knowledge of cartilage biology and pathology, including what is known about the relative contribution of collagenases and aggrecanases to cartilage degradation, the need to regulate the chondrocytic phenotype and the putative role of chondrocyte hypertrophy in the pathogenesis of degenerative and rheumatic joint disease. Recent advances in cartilage tissue engineering are also reviewed.
Articular cartilage is a unique and unusual tissue in which a single resident cell type, the chondrocyte, is responsible for the synthesis, maintenance and degradation of its extensive extracellular matrix. Despite these diverse responsibilities, there are relatively few chondrocytes within cartilage, and combined with the fact that cartilage has no blood vessels and no innervation, cartilage is also a tissue that is poorly equipped for self-repair. Cartilage is predominantly extracellular matrix, and the properties of the matrix dominate its functional and biophysical properties. Destructive change to articular cartilage is one feature of degenerative and rheumatic joint diseases and, accordingly, therapies to limit these changes are needed to manage better patient outcomes. Although the underlying mechanisms driving degenerative and rheumatic diseases are distinct, and although cartilage plays a more active role in osteoarthritis (OA) compared with rheumatoid arthritis (RA), the signals that regulate chondrocyte biology, behaviour and bioactivity are overlapping. Cytokines, adipokines, inflammatory mediators and matrix fragments are present in cartilage and/or the synovial joint in both conditions, and are thought to affect chondrocyte physiology in a similar manner. Joint loading in synergy with molecular and biomechanical signals can further enhance joint degeneration, so it is not unreasonable to believe that disease-induced chondrocyte activities might show some similarities between OA and RA.
OA research traditionally has a strong focus on cartilage biology, even though the entire joint and subchondral bone is involved and despite continuing debate on the relative importance of cartilage changes, compared with bone changes in the disease process. By contrast, chondrocytes are thought to have a passive, less responsive role in the rheumatic diseases and have therefore received less attention than synovial and inflammatory cells in these conditions. This chapter therefore focusses on gaps, and recent developments, in our understanding of cartilage biology in degenerative diseases and considers how these findings might apply to rheumatic diseases. Insights gained into chondrocyte biology and pathology might lead to therapeutic approaches for both conditions.
Among the many questions relating to cartilage biology in degenerative and rheumatic diseases, the most fundamental one is the uncertain role of cartilage in the disease process: do chondrocytes drive cartilage pathology or do they merely respond to disease processes initiated from elsewhere in the joint? If cartilage is a passive responder to other pathological drivers, then repairing damaged cartilage (presuming that were possible) would be of little enduring value to the patient if the underlying disease processes remained unchecked. On the other hand, if cartilage changes contribute to the disease, which ones are the most important for therapeutic targeting? Is it valid to focus on specific molecular events such as induction of destructive proteinases or inflammatory mediators, or would modifying the entire cellular phenotype be a better strategy? The answers might differ between degenerative and rheumatic diseases, and likely even between patients and disease subgroups within each form of arthritis. Nevertheless, the answers will help guide new approaches to prevent, treat and reverse tissue damage in joint disease.
Will aggrecanase or collagenase inhibitors best protect against cartilage erosion in degenerative and rheumatic joint diseases?
The predominant collagen in adult articular cartilage is the fibrillar type II collagen, a homotrimer of α1(II) chains that assembles with collagen types IX and XI, extracellularly, to form heterofibrils. The type II collagen network gives cartilage its shape and tensile strength and provides a framework to resist the swelling pressure of aggrecan. Aggrecan swells because its many negatively charged glycosaminoglycan side chains attract counter ions, and consequently water, to create large hydrodynamic domains and high osmotic swelling pressure. In functional cartilage, the swelling pressure of aggrecan is balanced by tension within the collagen scaffold; proteolysis of either matrix component disturbs this balance and compromises the ability of cartilage to redistribute compressive forces.
Our understanding of which proteinases are the key drivers of cartilage degradation, and their modes of action and regulation, is incomplete despite decades of research endeavour that has led to important advances. For example, although it is now clear that matrix metalloproteinase (MMP)-13 (collagenase-3) is the major cartilage collagenase, and that a disintegrin and metalloproteinase with thrombospondin motifs (ADAMTS)-4 and/or −5 are the main aggrecanases in human cartilage, it is not at all clear how these enzymes are activated from their zymogen forms, nor precisely how their activities are modulated in vivo . It is also not clear whether collagenases or aggrecanases are responsible for initiating and then progressing cartilage erosion. Accordingly, one key question that remains unanswered is whether the most appropriate cartilage-protecting therapies would be collagenase inhibitors or aggrecanase inhibitors.
It has been impossible to address this question experimentally using the traditional agents and models that are used to explore cartilage degradation in vitro . At early times in culture, cartilage explants treated with catabolic agents typically show loss of aggrecan fragments into the medium and negligible, if any, collagen loss. At later time points, after most aggrecan has been degraded and released from the tissue, collagenases cleave collagen II at the primary cleavage site, followed by denaturation and secondary cleavage that precedes loss of collagen fragments into the medium. This pattern of early aggrecanolysis followed by later collagenolysis has been described for cartilage explants from several species, following treatment with catabolic agents including interleukin-1 (IL-1), tumour necrosis factor-α (TNFα), oncostatin M and retinoic acid. Thus, while established methods for promoting cartilage breakdown allow us to independently stimulate aggrecan release over short time periods, they do not allow us to independently stimulate collagenolysis. For this reason, experiments to investigate the cartilage-sparing effects of aggrecanase inhibitors, compared with collagenase inhibitors, have not been feasible.
Aggrecanolysis in cartilage degradation
Historically, aggrecanolysis was thought to be the initiating event in pathological cartilage erosion. This was largely because histological evidence of aggrecanolysis, detected by a loss of metachromatic staining, occurs well before the clinical or radiographic symptoms of disease are visible. This characteristic loss of metachromatic staining prior to cartilage erosion is highly reproducible across species, experimental models and clinical samples. A defining study linking deterioration in cartilage function with aggrecan loss was reported in the 1950s . This study showed that injection of an aggrecan-degrading enzyme into an upright rabbit ear is sufficient to destroy cartilage turgor and produce floppy ears, even though the collagen network is resistant to the proteinase and remains intact. In the same study, the rabbit ears regained their turgor within 3–4 days, showing not only that aggrecan depletion is reversible, but also that restoration of aggrecan content can restore the functional properties of the tissue.
Further support for the view that preventing aggrecanolysis is the best strategy for preventing cartilage erosion comes from a study showing that although a selective aggrecanase inhibitor blocked IL-1-induced aggrecanolysis in cultured cartilage explants, the inhibitor also blocked collagen degradation . To eliminate the possibility of a non-specific effect of the inhibitor on collagenase activity, the investigators added the inhibitor to the cultures at day 6, after aggrecan was depleted, and found that the inhibitor did not prevent collagen degradation . These results support the idea that aggrecan protects collagen from collagenase attack, possibly via direct steric blockade by chondroitin sulphate chains. The authors postulated that collagen II fibrils, preferentially bound to aggrecan at the keratan sulphate (KS)-rich region , would be protected from collagenases by the volume exclusion effect of aggrecan’s chondroitin sulphate-rich region ( Fig. 1 ).

Collagenolysis in cartilage degradation
On the flip side of the discussion above is the view that collagenolysis (not readily detected by simple histological stains) precedes aggrecan loss. Maroudas first championed the idea that clipping of the collagen network was sufficient to initiate cartilage damage, based on her observations that cartilage degeneration in osteoarthritic disease is accompanied by swelling and increased water content . This might seem counterintuitive given that cartilage degeneration is usually accompanied by loss of aggrecan (and hence water), but Maroudas proposed that clipping, and thereby weakening of the collagen network, would release the elastic restraint on aggrecan, allowing it to swell further and to occupy even greater hydrodynamic domains . In support of this model, other investigators have identified damaged, unwound collagen II colocalised with regions of aggrecan loss and concluded that aggrecanases gain access to aggrecan substrate as a consequence of collagen clipping ( Fig. 1 ). It is easy to envisage a scenario whereby localised collagen clipping might occur at the cartilage surface, causing aggrecan loss, which then facilitates proteinase access to the collagen fibres, causing a cascade of aggrecan and collagen proteolysis from the superficial zone into the deeper zones. Indeed, it is interesting to speculate that type I collagen, resident in the cartilage superficial zone, might be susceptible to a wider range of collagen-degrading enzymes than type II collagen, and also that during arthritic disease, a greater variety and abundance of collagen-degrading enzymes or collagenase activators might be delivered to the cartilage surface by invading pannus, inflammatory cells, or secreted from the synovium or the infrapatellar fat pad.
It is interesting to note that many features of collagenolysis and aggrecanolysis are diametrically opposed ( Table 1 ). Type II collagen has evolved as a protein that is highly resistant to proteinase attack and with an extraordinarily long half-life, consistent with the fact that collagenolysis is essentially irreversible. By contrast, since aggrecan loss is readily reversible , there has been less evolutionary pressure to protect it from proteolysis and to preserve its residence time in cartilage. 1
1 MMP-1, -2, -8, -13, -14 and cathepsin K can degrade fibrillar collagens.
Aggrecan | Collagen II |
---|---|
Readily degraded by many proteinases under physiological conditions | Degraded only by collagenases under physiological conditions |
Aggrecanases cleave at multiple sites in the aggrecan core protein | The initiating collagenase cleavage is at a single site in the collagen II triple helix |
IL-1 stimulation induces active aggrecanase in cartilage; aggrecanases are constitutively active in synovium | IL-1 stimulation induces latent collagenase in cartilage |
Extensive aggrecanolysis is reversible in vivo | Extensive collagenolysis is not reversible in vivo |
Half-life in cartilage is ∼3.4 years | Half-life in cartilage is ∼117 years |
In considering the in vitro examples of stimulated cartilage explants in which collagenolysis does not occur until late, the ‘when’ question is most intriguing. Collagenolysis can be induced earlier if exogenous activators are added to the system , suggesting that enzyme activation is the rate-limiting step in collagenolysis, but what then is the initiator that tips the balance in favour of MMP activation and why is it delayed? Indeed, there is likely to be more than one initiator.
How are collagenases activated?
Collagenases and aggrecanases are synthesised as zymogen precursors that remain inactive until their prodomains are removed by proteolysis, via unknown activators. Several serine proteinases are candidates for these activating roles, but pinpointing exactly which ones are involved in cartilage remains a challenge. Based on their activities in vitro , these serine proteinases are thought to act indirectly, by activating MMP-3 (stromelysin) and MMP-2 (gelatinase A), which can subsequently activate collagenases (reviewed in ).
The matriptase-1 story is a new and interesting one . Matriptase-1 is a transmembrane serine proteinase comprising an N-terminal transmembrane domain with multiple extracellular domains including the catalytic domain. Recombinant matriptase-1 can activate pro-MMP-1 and pro-MMP-3 in the test-tube, but it cannot directly activate pro-MMP-13. Although matriptase-1 expression is not detectable in normal cartilage , it is substantially elevated in human OA cartilage . More importantly, the addition of exogenous matriptase-1 to human OA cartilage induces collagenolysis in cartilage explant cultures without the need for cytokine stimulation. This collagenolysis was blocked by a metalloproteinase inhibitor, suggesting a role for matriptase-1 in the direct activation of MMP-1 and MMP-3, and subsequent activation of MMP-13 by MMP-3. Matriptase-1 is unusual for a serine proteinase in that it is self-activating . However, if matriptase is the key initiator of collagenase activation, it is still unclear what spatiotemporal relationship it might have with the MMP-1 and MMP-3 intermediaries, that accounts for the lengthy delays seen in collagenolysis.
Another putative activator of collagenases in cartilage is Activated Protein C (APC). APC, more commonly known for its anticoagulant role in plasma, is derived from Protein C, bound to the endothelial protein C receptor (EPCR), in a mechanism involving thrombin bound to thrombomodulin . Human and ovine articular chondrocytes express messenger RNA (mRNA) for protein C, EPCR and thrombomodulin, suggesting that chondrocytes have the necessary machinery for APC activation . In ovine cartilage explant cultures, the addition of exogenous APC to IL-1- or TNFα-treated samples stimulates collagenolysis via an MMP-mediated mechanism, without increasing the levels of MMP mRNA or decreasing the levels of tissue inhibitor of metalloproteinase (TIMP) mRNA . Rather, the addition of exogenous APC increased the levels of active MMP-2 and MMP-9. APC did not directly activate pro-MMP-13, but since collagenolysis was increased in cytokine-treated cartilage explants, the data suggest a mechanism whereby APC activates pro-MMP-2, which in turn can activate pro-MMP-13.
Some serine proteinases with potential to initiate MMP activation cascades are present in arthritic joints, but not expressed by chondrocytes. Plasmin, derived from plasminogen, is a good example, and has a long history of guilt by association with respect to collagenase activation in arthritic joints. Other cells in the synovial joint express plasminogen, and since the plasminogen activators, urokinase-type plasminogen activator (uPA) and tissue-type plasminogen activator (tPA), are expressed at the chondrocyte membrane, there is potential for plasmin activity to also exist at the chondrocyte cell surface where collagenolysis occurs . However, the possible role of plasmin in the collagenase activation cascade in cartilage is complicated by the fact that plasmin activation of MMP-13 in vitro leads to loss of the MMP-13 haemopexin domain, and therefore loss of its collagenolytic activity .
Other potential collagenase-activating enzymes in cartilage are the mast cell tryptases and chymases. These serine proteinases are released during mast cell degranulation and tryptase-positive mast cells are increased in joints of patients with RA and OA . Mast cell tryptase can be immunodetected at sites of cartilage erosion, often colocalised with collagenase and MMP-3, at the cartilage–pannus junction . In vitro , chymase can activate pro-MMP-1 and pro-MMP-3 and tryptase can activate pro-MMP-3 which, subsequently, can activate MMP-9 . Whether mast cell serine proteinases have a role in vivo is unknown. Although there are good data showing that mast cells and their cache of MMP-activating enzymes are present at the right place, at the right time, and in vivo data showing that mice lacking the orthologue of human tryptase-β1 have diminished cartilage erosion in the methylated bovine serum albumin/interleukin-1β (mBSA/IL-1β)-induced model of inflammatory arthritis , the evidence directly implicating tryptase/chymotryptase involvement will be difficult to obtain.
How are aggrecanases activated?
In contrast to the collagenases, the aggrecanases are directly activated by members of the proprotein convertase (PC) family members, which are also serine proteinases. PC enzymes remove the aggrecanase propeptide, cleaving at the R/KX n R/K↓ consensus motif, where X is any amino acid and n = 0, 2, 4 or 6. There are seven mammalian PCs that recognise this motif, some of which are membrane bound (furin, PC5/6B and PC7), while others are found in the dense core granules (PC1/3, PC2 and PC5/6A) and/or secreted to the extracellular space (PC4, PACE4 and PC5/6A) (reviewed in ). Furin is also found at the plasma membrane or in a shed, soluble form . It is highly likely that aggrecanase activation is spatially controlled, but teasing out which PC cleaves which aggrecanase, and the cellular location at which the cleavage takes place has proved a challenge. For example, studies in cell lines have shown that furin activates ADAMTS-4 in the trans-Golgi network , but activates ADAMTS-5 in the extracellular matrix (ECM) and activates a putative aggrecanase, ADAMTS-9, at the cell surface . Other in vitro studies have shown that PACE4 and PC5/6 activate ADAMTS-4 , and PC7 can activate ADAMTS-5 . Perhaps the most definitive study has been done by Malfait and colleagues, who identified PACE4 as the major PC activity in human osteoarthritic cartilage. Addition of recombinant PACE4 to osteoarthritic cartilage or bovine cartilage in culture increased the release of aggrecan fragments, and also the generation of ADAMTS-4 and ADAMTS-5 lacking their prodomains, consistent with the activation of latent ADAMTS-4/-5 in situ .
How do articular and growth plate cartilages differ?
Cartilage has two major roles in the human body, and chondrocytes adopt specific phenotypes for each of them. Mature articular cartilage is designed to last and function for an entire lifetime, so the resident cells have a slow turnover rate and normally display a stable phenotype . It is the articular cartilage that is compromised in adult joint diseases. By contrast, growth plate cartilage present in the developing skeletal anlagen forms a template for subsequent endochondral bone formation and longitudinal bone growth. During this process, growth plate chondrocytes enter a highly regulated progression through phases of proliferation, differentiation and degeneration . The terminal differentiation of growth plate chondrocytes is accompanied by a large increase in cell volume and is therefore termed ‘hypertrophy’. Chondrocyte hypertrophy, together with the preceding proliferation of cells and increasing matrix production, is the main driver of bone growth and is tightly controlled by endocrine and local signals. Importantly, because growth plate cartilage is destined to be replaced by bone, hypertrophic chondrocytes die during this replacement process, in marked contrast with the fate of articular chondrocytes that ideally last a lifetime. In addition, hypertrophic chondrocytes secrete proteins that assist with breakdown of the cartilage matrix (for example, MMP13), attract invading blood vessels (for example, vascular endothelial growth factor (VEGF)) and control the differentiation of osteoblasts and osteoclasts (for example, Indian hedgehog, receptor activator of nuclear factor-kappaB ligand (RANKL)) . Thus, the biochemical profile and the ultimate fate of hypertrophic chondrocytes contrast starkly with those of adult articular chondrocytes in the maintenance of a stable cartilage ECM.
There are important questions in cartilage biology, around the relationship between articular and growth plate chondrocytes, that deserve further consideration. For example, do articular and growth plate chondrocytes share the same embryonic and developmental origins? Lineage tracing experiments indeed suggest a different origin , but the exact details are unknown. What are the common features and, equally important, what are the distinguishing features of articular and growth plate chondrocytes? What are the key signalling pathways, transcription factors and/or epigenetic events that dictate the distinctly different cellular phenotypes of articular and growth plate chondrocytes? Finally, is switching from an articular phenotype to a growth plate phenotype, or vice versa, part of the disease process and can the switch be manipulated for therapeutic purposes?.
These questions impact directly on the challenge of tissue engineering for cartilage regeneration. Successful regeneration of articular cartilage requires not only detailed knowledge of the molecular mechanisms that determine chondrocyte cell fate, but also the appropriate tools to navigate the cells towards a stable articular phenotype, as opposed to a growth plate phenotype that ultimately leads to cartilage resorption and death of the chondrocytes themselves . Furthermore, numerous studies over the past two decades have reported the ectopic initiation of chondrocyte hypertrophy in both human OA and in animal models of OA. Markers thought to be selective for hypertrophic chondrocytes, and not usually expressed in articular chondrocytes, have been detected in articular cartilage from OA patients and in animal models of OA, including collagen X, alkaline phosphatase, osteocalcin and CD36 . Along with these molecular markers, increased formation of basic calcium phosphates (another feature of terminal hypertrophic cartilage) has been documented as a consistent feature of human OA cartilage .
Does chondrocyte hypertrophy contribute to the pathogenesis of degenerative and rheumatic joint disease?
While these data suggest that articular chondrocytes can change their phenotype to one resembling hypertrophic growth plate cells, the role and pathological importance of these changes in OA progression cannot be resolved using human cartilage explants, typically obtained from joints at late stages of disease. However, the results of studies with animal models, and in particular genetically modified mouse strains, provide evidence that inhibiting chondrocyte hypertrophy can protect against OA progression. For example, several transcription factors including Runx2, CCAAT-enhancer-binding protein β (C/EBPβ) and hypoxia-inducible factor 2α (HIF-2α) promote chondrocyte hypertrophy during development. Mice with deletions for one or both alleles encoding these factors have been examined in surgical models of OA . Strikingly, complete or partial loss of either one of these transcription factors significantly slows progressive cartilage erosion, suggesting that preventing hypertrophic differentiation of chondrocytes indeed protects against OA pathology, at least in these experimental models.
Much less is known about potential hypertrophic changes in articular chondrocytes in rheumatic diseases. Since the underlying mechanisms are fundamentally different, this might not be surprising. However, it is worth noting that inflammatory signalling can induce chondrocyte hypertrophy. For example, toll-like receptor (TLR) 2/4 ligands can induce hypertrophy through the signalling adapter MyD88 . Similarly, several cytokines including TNFα and IL-8 can promote chondrocyte hypertrophy through receptor for advanced glycation end products (RAGE)-dependent pathways . Furthermore, native collagen fragments might influence chondrocyte differentiation and hypertrophy based on evidence that an MMP-13 inhibitor (RS-102481 ) can prevent differentiation of prehypertrophic chondrocytes and block mineralisation, type X collagen expression and Runx2 expression, in vitro . These results suggest that there is certainly potential for inducing chondrocyte hypertrophy in rheumatic diseases and, indeed, hypertrophic changes were reported in a lipopolysaccharide-induced form of synovitis in horses . However, in general, the role and incidence of chondrocyte hypertrophy in the inflammatory arthritides is not well understood.
Beyond questions regarding the fundamental differences in cartilage between rheumatic and degenerative joint diseases, are questions regarding the phenotypic switch from articular to growth plate chondrocytes . How similar is ectopic hypertrophy in articular chondrocytes to the hypertrophy process that characterises endochondral ossification? Note that the name-giving feature of hypertrophy, the large increase in cell volume, is not usually seen in OA (or only to a minimal degree), suggesting that the term ‘chondrocyte hypertrophy’ encompasses molecular and cellular variations. How prevalent is hypertrophy in human OA (and RA), and is it associated with particular disease sub-types (for example, post-traumatic OA which many animal models try to recapitulate)? Is hypertrophy a driver or a consequence of pathological change, or a failed attempt at cartilage repair? Can results from the surgical models of OA in mice be applied to understanding human OA, given the differences in skeletal structure, locomotion patterns and aetiology? In terms of cartilage repair, is it possible to promote proliferation of articular chondrocytes without triggering the entire growth plate pathway leading to hypertrophy? While chondrocyte hypertrophy appears to be involved, at least in some forms of OA, a clear definition of the term ‘OA hypertrophy’ is lacking.
Which molecular pathways determine chondrocyte identity?
Irrespective of whether future studies confirm a major role for chondrocyte hypertrophy in degenerative or rheumatic joint diseases, it will be important to delineate the molecular mechanisms that keep the two lineages apart. For example, tissue engineering of articular cartilage requires approaches that promote formation of articular chondrocytes but inhibit hypertrophic differentiation. Relatively little is known about these mechanisms. Growth factors including transforming growth factor β (TGFβ), hedgehog and Wnt proteins are thought to be involved . TGFβ signalling is of particular importance since overexpression of a dominant-negative TGFβ receptor, or deletion of the downstream Smad 3 gene, both induce ectopic hypertrophy in articular chondrocytes . These results suggest that the classical TGFβ pathway, operating via Smad2/3, maintains the articular cartilage phenotype. Note, however, that a switch in the receptors for TGFβ signalling in ageing cartilage is thought to change the function of this pathway from protective to destructive .
In addition to these pathways, the Ets family member ERG, and in particular its splice variant C-1-1, has been identified as a key transcription factor that maintains articular chondrocyte identity and represses the growth plate phenotype in mice and chickens . There is presently no data describing ERG’s potential role in degenerative or rheumatic diseases.
Which epigenetic factors control the chondrocyte phenotype?
A strong argument has recently been made for the role of epigenetic factors in controlling chondrocyte identity , even though the exact epigenetic players and mechanisms involved are unknown. Epigenetic mechanisms are thought to control gene expression through at least three different mechanisms: DNA methylation, histone modifications and non-coding RNAs. Limited knowledge on how these mechanisms control the chondrocyte phenotype is available ( Fig. 2 ), but much remains to be learned.
Inhibiting DNA methylation can induce early chondrogenic differentiation (from precursor cells to chondrocytes) as well as hypertrophic differentiation of chondrocytes in vitro . These results indicate that DNA methylation suppresses the expression of key genes that drive both early and late chondrocyte differentiation. However, the identity of these genes is unknown and the mechanism is more complex because other genes show the reverse regulation; in other words, increased methylation is associated with a more mature chondrocyte phenotype. Moreover, methylation of promoters of several genes with key functions in cartilage, including superoxide dismutase 2 (SOD2), ADAMTS-4, MMP13 and bone morphogenetic protein-7/osteogenic protein-1 (BMP-7/OP-1), is altered in OA or cartilage ageing . These data suggest that differential DNA methylation can drive changes in the cellular phenotype and/or gene expression in chondrocytes, but little is known about how these events are controlled and whether they are causatively linked to disease progression in degenerative or rheumatic diseases.
Similarly, our understanding of the role of histone modification in cartilage growth, development, physiology and pathology is very limited. Mice lacking the histone deacetylase, HDAC4, show accelerated hypertrophic differentiation of growth plate chondrocytes , and haploinsufficiency for HDAC4 causes brachydactyly in humans . However, whether HDAC4 elicits a phenotypic switch between articular and growth plate chondrocytes in OA or RA has not been shown. More work has been done on the role of another histone deacetylase, SirT1, in articular cartilage and in OA. SirtT1 activity is directly regulated by nicotinamide adenine dinucleotide (NAD) and therefore closely linked to cellular and body metabolism. Since metabolic influences are increasingly recognised as key regulators of OA progression, SirT1 is a promising candidate for functional studies. SirT1 promotes chondrogenic gene expression and protects chondrocytes from apoptosis . In addition, knockdown of SirT1 expression increases the expression of proteinases and the hypertrophy marker collagen X, suggesting that this gene could be an important regulator of aberrant hypertrophic differentiation in articular cartilage. Interestingly, TNFα induces cathepsin B-mediated degradation of SirT1 in chondrocytes and could plausibly mediate loss of SirT1 in both degenerative and rheumatic joint conditions . Paradoxically, another report shows that IL-1β activates SirT1 and that a dominant-negative version of SirT1 blocks the IL-1β-induced dedifferentiation of articular chondrocytes . Further studies are required to connect these seemingly contradictory scenarios and to examine the role of SirT1 in OA and RA pathogenesis using in vivo models. From a wider perspective, the many proteins that control acetylation, methylation, phosphorylation and other histone modifications in cartilage biology and pathology represent a wide-open field for future studies with potential clinical significance.
Non-coding RNAs (ncRNAs) are the third branch of epigenetic regulators. They have received somewhat more attention in recent years, although the focus has been mainly on the micro (mi) RNAs that act by suppressing specific target gene expression, usually with several genes targeted by the same miRNA. Cartilage-specific deletion of the gene encoding Dicer, a key component of miRNA synthesis, leads to severe defects in skeletal development ; early lethality precluded arthritis studies in these mice. One miRNA, miR-140, is specifically expressed in cartilage , under the control of the chondrogenic transcription factor Sox9 and has important roles in both cartilage development and arthritis. Deletion of the miR-140 gene leads to reduced bone growth, caused most likely by decreased chondrocyte proliferation and/or accelerated hypertrophy . Furthermore, miR-140-deficient mice show accelerated OA-like degeneration of articular cartilage, whereas overexpression of miR-140 protects against disease progression in the antigen-induced model of inflammatory arthritis, suggesting that this specific gene has potential cartilage-protective effects in both degenerative and rheumatic arthritides. It is interesting that ADAMTS-5 is one of the genes suppressed by miR-140 ; additional targets such as HDAC4, MMP13 and the peptidase Dnpep have also been described . Collectively, these findings suggest a complex role for miR-140 since it appears to suppress markers of hypertrophy such as MMP13, as well as repressors of hypertrophy such as HDAC4. The miRNA miR-146a expressed in cartilage, synovium and glial cells is another miRNA involved in OA pathology and additional miRNAs identified in large-scale screens in cartilage and OA models await functional characterisation. Similarly, many miRNAs have been implicated in rheumatic diseases, although the focus of these studies is mostly on synovial and inflammatory cells rather than cartilage . Overall, there is strong evidence that miRNAs play important roles in cartilage development, homeostasis and pathology, and a high probability that many more are yet to be discovered.
Other changes in chondrocyte phenotype
In addition to becoming more growth plate-like, there are other changes to chondrocyte phenotype and physiology that potentially contribute to degenerative and rheumatic diseases. One example is chondrocyte dedifferentiation, whereby chondrocytes acquire a fibroblast-like phenotype marked by changes in cell shape, cytoskeletal organisation and pattern of ECM molecule expression. Most dedifferentiation studies have been done in vitro , often in monolayer culture which in itself induces a loss of chondrocyte phenotype; however, there is evidence suggesting that dedifferentiation changes observed in vitro might be relevant for pathology in vivo . For example, the expression of transforming growth factor alpha (TGFα), a member of the epidermal growth factor family, is increased in an in vivo model of rat OA and in cartilage of some OA patients . TGFα is also found in the synovium and synovial fluid of patients with RA , where it has the potential to act directly on chondrocytes. In vitro TGFα induces several features of chondrocyte dedifferentiation including actin stress fibre formation and down-regulation of Sox9, collagen II and aggrecan expression. TGFα also induces expression of MMP-13, but without inducing hypertrophy or expression of other hypertrophic marker genes such as collagen X . Again, although the mechanisms that control these changes are incompletely understood, the data do suggest that the dedifferentiation changes observed in vitro are compatible with putative functions of TGFα as a mediator of disease progression in vivo .
How do articular and growth plate cartilages differ?
Cartilage has two major roles in the human body, and chondrocytes adopt specific phenotypes for each of them. Mature articular cartilage is designed to last and function for an entire lifetime, so the resident cells have a slow turnover rate and normally display a stable phenotype . It is the articular cartilage that is compromised in adult joint diseases. By contrast, growth plate cartilage present in the developing skeletal anlagen forms a template for subsequent endochondral bone formation and longitudinal bone growth. During this process, growth plate chondrocytes enter a highly regulated progression through phases of proliferation, differentiation and degeneration . The terminal differentiation of growth plate chondrocytes is accompanied by a large increase in cell volume and is therefore termed ‘hypertrophy’. Chondrocyte hypertrophy, together with the preceding proliferation of cells and increasing matrix production, is the main driver of bone growth and is tightly controlled by endocrine and local signals. Importantly, because growth plate cartilage is destined to be replaced by bone, hypertrophic chondrocytes die during this replacement process, in marked contrast with the fate of articular chondrocytes that ideally last a lifetime. In addition, hypertrophic chondrocytes secrete proteins that assist with breakdown of the cartilage matrix (for example, MMP13), attract invading blood vessels (for example, vascular endothelial growth factor (VEGF)) and control the differentiation of osteoblasts and osteoclasts (for example, Indian hedgehog, receptor activator of nuclear factor-kappaB ligand (RANKL)) . Thus, the biochemical profile and the ultimate fate of hypertrophic chondrocytes contrast starkly with those of adult articular chondrocytes in the maintenance of a stable cartilage ECM.
There are important questions in cartilage biology, around the relationship between articular and growth plate chondrocytes, that deserve further consideration. For example, do articular and growth plate chondrocytes share the same embryonic and developmental origins? Lineage tracing experiments indeed suggest a different origin , but the exact details are unknown. What are the common features and, equally important, what are the distinguishing features of articular and growth plate chondrocytes? What are the key signalling pathways, transcription factors and/or epigenetic events that dictate the distinctly different cellular phenotypes of articular and growth plate chondrocytes? Finally, is switching from an articular phenotype to a growth plate phenotype, or vice versa, part of the disease process and can the switch be manipulated for therapeutic purposes?.
These questions impact directly on the challenge of tissue engineering for cartilage regeneration. Successful regeneration of articular cartilage requires not only detailed knowledge of the molecular mechanisms that determine chondrocyte cell fate, but also the appropriate tools to navigate the cells towards a stable articular phenotype, as opposed to a growth plate phenotype that ultimately leads to cartilage resorption and death of the chondrocytes themselves . Furthermore, numerous studies over the past two decades have reported the ectopic initiation of chondrocyte hypertrophy in both human OA and in animal models of OA. Markers thought to be selective for hypertrophic chondrocytes, and not usually expressed in articular chondrocytes, have been detected in articular cartilage from OA patients and in animal models of OA, including collagen X, alkaline phosphatase, osteocalcin and CD36 . Along with these molecular markers, increased formation of basic calcium phosphates (another feature of terminal hypertrophic cartilage) has been documented as a consistent feature of human OA cartilage .
Does chondrocyte hypertrophy contribute to the pathogenesis of degenerative and rheumatic joint disease?
While these data suggest that articular chondrocytes can change their phenotype to one resembling hypertrophic growth plate cells, the role and pathological importance of these changes in OA progression cannot be resolved using human cartilage explants, typically obtained from joints at late stages of disease. However, the results of studies with animal models, and in particular genetically modified mouse strains, provide evidence that inhibiting chondrocyte hypertrophy can protect against OA progression. For example, several transcription factors including Runx2, CCAAT-enhancer-binding protein β (C/EBPβ) and hypoxia-inducible factor 2α (HIF-2α) promote chondrocyte hypertrophy during development. Mice with deletions for one or both alleles encoding these factors have been examined in surgical models of OA . Strikingly, complete or partial loss of either one of these transcription factors significantly slows progressive cartilage erosion, suggesting that preventing hypertrophic differentiation of chondrocytes indeed protects against OA pathology, at least in these experimental models.
Much less is known about potential hypertrophic changes in articular chondrocytes in rheumatic diseases. Since the underlying mechanisms are fundamentally different, this might not be surprising. However, it is worth noting that inflammatory signalling can induce chondrocyte hypertrophy. For example, toll-like receptor (TLR) 2/4 ligands can induce hypertrophy through the signalling adapter MyD88 . Similarly, several cytokines including TNFα and IL-8 can promote chondrocyte hypertrophy through receptor for advanced glycation end products (RAGE)-dependent pathways . Furthermore, native collagen fragments might influence chondrocyte differentiation and hypertrophy based on evidence that an MMP-13 inhibitor (RS-102481 ) can prevent differentiation of prehypertrophic chondrocytes and block mineralisation, type X collagen expression and Runx2 expression, in vitro . These results suggest that there is certainly potential for inducing chondrocyte hypertrophy in rheumatic diseases and, indeed, hypertrophic changes were reported in a lipopolysaccharide-induced form of synovitis in horses . However, in general, the role and incidence of chondrocyte hypertrophy in the inflammatory arthritides is not well understood.
Beyond questions regarding the fundamental differences in cartilage between rheumatic and degenerative joint diseases, are questions regarding the phenotypic switch from articular to growth plate chondrocytes . How similar is ectopic hypertrophy in articular chondrocytes to the hypertrophy process that characterises endochondral ossification? Note that the name-giving feature of hypertrophy, the large increase in cell volume, is not usually seen in OA (or only to a minimal degree), suggesting that the term ‘chondrocyte hypertrophy’ encompasses molecular and cellular variations. How prevalent is hypertrophy in human OA (and RA), and is it associated with particular disease sub-types (for example, post-traumatic OA which many animal models try to recapitulate)? Is hypertrophy a driver or a consequence of pathological change, or a failed attempt at cartilage repair? Can results from the surgical models of OA in mice be applied to understanding human OA, given the differences in skeletal structure, locomotion patterns and aetiology? In terms of cartilage repair, is it possible to promote proliferation of articular chondrocytes without triggering the entire growth plate pathway leading to hypertrophy? While chondrocyte hypertrophy appears to be involved, at least in some forms of OA, a clear definition of the term ‘OA hypertrophy’ is lacking.
Which molecular pathways determine chondrocyte identity?
Irrespective of whether future studies confirm a major role for chondrocyte hypertrophy in degenerative or rheumatic joint diseases, it will be important to delineate the molecular mechanisms that keep the two lineages apart. For example, tissue engineering of articular cartilage requires approaches that promote formation of articular chondrocytes but inhibit hypertrophic differentiation. Relatively little is known about these mechanisms. Growth factors including transforming growth factor β (TGFβ), hedgehog and Wnt proteins are thought to be involved . TGFβ signalling is of particular importance since overexpression of a dominant-negative TGFβ receptor, or deletion of the downstream Smad 3 gene, both induce ectopic hypertrophy in articular chondrocytes . These results suggest that the classical TGFβ pathway, operating via Smad2/3, maintains the articular cartilage phenotype. Note, however, that a switch in the receptors for TGFβ signalling in ageing cartilage is thought to change the function of this pathway from protective to destructive .
In addition to these pathways, the Ets family member ERG, and in particular its splice variant C-1-1, has been identified as a key transcription factor that maintains articular chondrocyte identity and represses the growth plate phenotype in mice and chickens . There is presently no data describing ERG’s potential role in degenerative or rheumatic diseases.
Which epigenetic factors control the chondrocyte phenotype?
A strong argument has recently been made for the role of epigenetic factors in controlling chondrocyte identity , even though the exact epigenetic players and mechanisms involved are unknown. Epigenetic mechanisms are thought to control gene expression through at least three different mechanisms: DNA methylation, histone modifications and non-coding RNAs. Limited knowledge on how these mechanisms control the chondrocyte phenotype is available ( Fig. 2 ), but much remains to be learned.
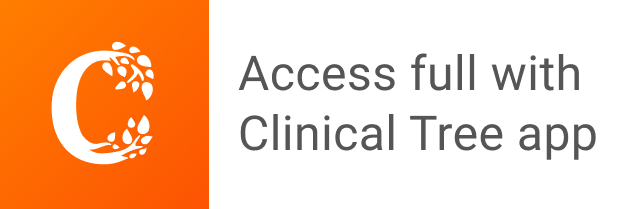