Fig. 19.1
Schematic overview of skeletal muscle structure
Skeletal muscle function is controlled by motor neurons that enter the muscle at the motor point. Each individual muscle fiber is contacted by axon terminals at a single neuromuscular junction [13, 22]. All muscle fibers innervated by one nerve axon along with the axon itself make up one motor unit that functions as a whole. The number of fibers per motor unit and the number of motor units vary between different skeletal muscles [13].
Skeletal muscle is richly vascularized. Blood supply to the muscle comes from feed arteries that enter the muscle tissue and continue to branch out further to the point of a network of capillaries (embedded in the endomysium) running alongside the muscle fibers [23].
19.3 Muscle Healing
Skeletal muscle tissue normally has a low turnover rate [24]. However, following injury, skeletal muscle tissue has the ability to initiate a rapid repair response. This response can be subdivided into several sequential yet overlapping phases: degeneration and inflammation, regeneration and remodeling [16–19, 25–27].
19.3.1 Degeneration and Inflammation
Excessive forces, whether direct (e.g., contusion due to blunt trauma) or indirect (e.g., strain injury due to excessive tensile forces), result in rupture of muscle fibers. Initially, the gap that is created by retraction of the ruptured fibers is filled with hematoma as a consequence of the rupturing of blood vessels that run parallel to the muscle fiber.
There is necrosis of a muscle fiber or muscle fiber segment, triggered by disruption of the plasma membrane followed by increased cell permeability, calcium influx, and activation of calcium-dependent proteases [15, 26, 28].
The injured tissue, activated platelets, and activated endothelial cells release factors that activate/recruit resident (in the epimysium/perimysium) and circulating inflammatory cells (leukocytes) [15, 29, 30]. First, neutrophils rapidly invade the damaged area, followed by monocytes/macrophages [15, 26, 31].
Neutrophils are believed to be involved in promoting muscle damage by proteolytic or oxidative modification of injured muscle tissue, in order to allow phagocytosis of the necrotic debris by neutrophils or macrophages [31, 32]. Neutrophils also release a variety of pro-inflammatory molecules to recruit/attract other inflammatory cells such as monocytes/macrophages [27].
Initially, the recruited macrophages present an inflammatory profile to contribute to the degeneration and inflammation process and secrete pro-inflammatory cytokines [25, 26]. However, macrophages are able to adapt to the changing microenvironment, and their different activation states have been associated with different (in vitro) phenotypes and functions [29, 33]. Several days after recruitment, they undergo a phenotype switch to an anti-inflammatory profile and play an active role in promoting muscle regeneration [26, 29, 33, 34].
19.3.2 Regeneration and Remodeling
The regenerative phase is characterized by two concurrent processes: regeneration of muscle fibers and the formation of connective (scar) tissue.
Satellite cells play a vital role in the muscle regeneration process. Under normal circumstances, satellite cells are in a quiescent state (i.e., no cell cycling). Following muscle injury, these quiescent satellite cells become activated by various stimuli, migrate to the site of injury, and re-enter the cell cycle in order to give rise to myogenic precursor cells (myoblasts). Finally, they will fuse together to form multinucleated myotubes or fuse with existing damaged muscle fibers [14, 35].
Revascularization by means of capillary ingrowth is essential for successful muscle healing, and is reported to be one of the first signs of muscle regeneration [16, 27].
The formation of connective (scar) tissue occurs simultaneously [20]. A primary matrix formed between the stumps of the ruptured muscle fibers by blood-derived fibrin and fibronectin is quickly invaded by fibroblasts [20, 27]. The aforementioned anti-inflammatory macrophages do not only produce anti-inflammatory cytokines, but also release pro-fibrotic factors such as TGF-β (Transforming Growth Factor-β) that activate fibroblasts [29, 36]. Once activated, these cells produce both extracellular matrix components (including collagen) and remodeling factors [36]. This increases the tensile strength of the primary scar tissue [20].
Initially, the regenerating muscle fibers adhere to the extracellular matrix at the lateral sides while they extend out of the preserved basement membrane and penetrate the scar tissue between the stumps of the ruptured muscle fibers [18, 20]. Subsequently, mini-MTJs are formed at the ends of the new muscle fibers, and the scar tissue between the muscle fiber stumps is reorganized and diminishes in size [17, 20]. Ultimately, the muscle fibers undergo maturation and sprouting axons may penetrate the scar tissue. These induce the formation of new neuromuscular junctions, since reinnervation is essential for muscle healing (particularly maturation [27]) and restoration of functional capacity [18, 20, 22].
19.4 Emerging Approaches
19.4.1 Platelet-Rich Plasma
There is growing interest in sports medicine and athletic communities in “regenerative medicine” using endogenous growth factors directly into the injury site to facilitate healing after injury [37, 38]. The most popular is probably the injection of platelet-rich plasma (PRP) [37, 38]. Platelets release various growth factors upon activation that are assumed to provide regenerative benefits.
PRP is derived from autologous whole blood using centrifuge separation systems to separate the platelet-rich plasma from other blood components. There are a multitude of autologous platelet-rich blood products commercially available that differ in their preparation procedure and cellular composition, such as platelets, growth factors, leukocytes, red blood cells and different cytokines [39, 40]. Generally, between 8 and 30 mL of whole blood is collected to obtain 3–4 mL of PRP. There is a wide variation in the ability of different preparation procedures to concentrate platelets from whole blood: from only a minor increase up to 20 times. Leukocyte concentrations can be reduced or increased, often referred as leukocyte-poor and leukocyte-rich PRP, respectively. Although often superiority is claimed of one PRP product over others, it remains unproven whether the composition of the PRP is relevant for the efficacy of PRP treatments, and this is the subject of ongoing debate in the literature.
PRP has been studied for a number of musculoskeletal disorders [37, 38]. Since the World Anti-Doping Agency permitted the intramuscular injection of platelet-rich plasma (PRP) in 2011, this experimental treatment has been increasingly used to treat acute muscle injuries in athletes [41]. The growth factors released are assumed to stimulate myoblast proliferation and accelerate muscle fiber regeneration.
Basic science studies have shown that myoblasts can be proliferated by growth factors like platelet-derived growth factor (PDGF), insulin-like growth factor (IGF-1), basic fibroblast growth factor (bFGF-2), and nerve growth factor (NGF) [42–45]. In deliberately injured animal muscles, these growth factors increase regeneration [42–45]. Whether the ratio of growth factors in PRP is appropriate for muscle healing in humans remains unproven.
Despite the promising results from basic research, and apparent widespread clinical use, a recent meta-analysis with pooled data of three randomized controlled trials (RCTs) [46–48] showed no superiority of PRP in treating acute hamstring muscle injuries on the time to return to play and the re-injury rate [49]. Since the publication of this systematic review, one additional RCT has been published, which showed a shortened time to return to play in the PRP-treated group compared to a control group that received no injection [50]. Unfortunately, this study is at risk for bias due to a lack of a placebo or any attempt to mask the lack of injection allowing for a placebo effect among patients. This study also found no effect on the re-injury rate.
There is one RCT that investigated the effect of PRP on muscle injuries other than hamstring injuries [51]. This study included 68 gastrocnemius and 3 rectus femoris injuries and showed no superiority of PRP over placebo on the time to (painless) return to pre-injury activities.
As there are currently no RCTs available of PRP treatment in other muscle injuries, it remains unknown to what extent the lack in efficacy of PRP can be generalized. However, as biology of muscle healing is generally consistent among skeletal muscle tissue, we argue that superiority of PRP treatment in other muscle injuries is not expected.
Our current scientific knowledge about PRP remains at a basic level, and there are many unanswered questions regarding its use in muscle injury [52]. These include some very fundamental questions, such as what concentration and ratio of growth factors are required for optimal muscle healing? Which specific growth factors are active? Is timing and number of injections important? Does the injected PRP remain at the injection site? Is the presence of leukocytes in the PRP beneficial or detrimental for muscle healing? There is currently no known “optimal” PRP content, nor is there a generally accepted protocol for PRP use with regard to volume, number, and timing of injections. It is unknown whether these factors will affect clinical outcome, as it has never been examined and compared. This requires further investigation in high-quality clinical trials.
In conclusion, considering the lack of efficacy in high-level RCTs and the many unanswered questions regarding its use, we currently discourage PRP treatment in muscle injuries.
19.4.2 Actovegin®
PRP is not the only blood product that is used in the treatment of muscle injury. Actovegin®, which is deproteinized hemodialysate of ultrafiltered calf serum, has recently been shrouded in controversy: Actovegin® was accused of being a performance-enhancing drug due to its alleged oxygen-carrying capacity [53]. However, WADA (World Anti-Doping Agency) has not added Actovegin® to the prohibited substances list since their analysis revealed it does not contain prohibited hormones nor blood cells susceptible to increase oxygen transport. Moreover, the active ingredient(s) of Actovegin® have yet to be identified, and therefore the exact mechanism of action remains elusive.
Systematic reviews on Actovegin® agreed that limited evidence exists for a positive effect of intramuscular Actovegin® injection [53, 54]. Both reviews included a study in which subjects with MRI-confirmed injuries were treated with a rehabilitation protocol or a rehabilitation protocol and three (2 mL) intramuscular Actovegin® injections [55]. Athletes with grade I injuries that were treated with Actovegin® injections returned to play significantly earlier (12 days on average, n = 4) than those that only received physiotherapy (20 days on average, n = 4). Unfortunately, as this was considered a pilot study there was no blinding and/or randomization, which increases the risk of bias. Future (randomized) studies including a placebo-group are necessary to determine whether Actovegin® injections improve muscle healing and speed up recovery. Until this high-quality evidence is obtained, we do not recommend it as a treatment for (acute) muscle injuries.
19.4.3 Traumeel®
Traumeel®, alone or in combination with Actovegin® [45], is another injection therapy that is used in the treatment of (acute) muscle injuries. Traumeel® is a homeopathic combination of diluted plant and mineral extracts [56], and is believed to have an anti-inflammatory effect [56]. Currently, there is no evidence on the effect of intramuscular injection of Traumeel® in (acute) muscle injury.
19.4.4 Glycosaminoglycans
In recent years, glycosaminoglycans (GAGs) have been widely evaluated as potential therapies for the treatment of musculoskeletal pathologies. Two of the most studied GAGs, the natural compounds chondroitin sulfate (CS) and glucosamine (GlcN), have demonstrated beneficial effects for the treatment of osteoarthritis both in preclinical studies [57–62] and in clinical trials [63–65]. Both CS and GlcN are considered symptomatic slow-acting drugs for osteoarthritis and have demonstrated clinical efficacy and safety when administered to OA patients, showing a synergistic effect when combined [64, 66, 67].
The mechanisms of action of CS and GlcN have been related to anti-inflammatory and anti-apoptotic effects [68–70]. It has been described that both CS and GlcN can inhibit the nuclear translocation of NF-κB, then preventing the transcription of pro-inflammatory cytokines [71–74]. CS seems to block NF-κB nuclear translocation and reverse the IL-1β-induced inflammatory activity by preventing the phosphorylation of ERK1/2 and p38MAPK [71, 75], whereas GlcN could exert NF-κB inhibition by preventing p50 and p65 protein migration to the nucleus [73].
With respect to the potential therapeutic effects of CS and GlcN for the treatment of skeletal muscle injuries, a recent preclinical study has evaluated the impact of CS and GlcN combination on muscle healing (data pending publication). In that study, a recently developed rat skeletal muscle injury animal model [76] which reproduces the muscle lesions seen in human athletes was used. The results showed that daily administration of both oral and intraperitoneal administration of CS and GlcN (combined) for 3 weeks induces not only an increase in intramuscular CS deposition in the injured area but also improves muscle force and stimulates the growth of regenerating muscle fibers. Although further investigation is required, these preclinical data suggests potentially positive effects of CS and GlcN administration for the treatment of skeletal muscle injuries in sports medicine.
19.4.5 Stem Cell Therapy
Similar to the use of growth factors, there is a lot of interest for the use of stem cells in muscle injuries. Stem cells comprise a population of undifferentiated cells that can undergo (asymmetric) cell division when activated by an appropriate stimulus, resulting in an identical stem cell (i.e., self-renewal) and a cell that is programmed to commit to a tissue lineage (i.e., differentiation). Thus, these cells have the ability to contribute to growth or regeneration, while simultaneously replenishing the stem cell pool [77, 78].
With respect to muscular disorders, the concept of transplanting cells with a high regenerative capacity as a treatment seems logical, and it has been explored for some time.
Early studies (late twentieth century) focused on transplantation of myogenic precursor cells (i.e., myoblasts, committed to development as a muscle cell) as a treatment for muscular dystrophy but results were limited due to, inter alia, immune rejection, poor survival, and limited spread of the transplanted cells [35, 77, 79, 80]. Thereafter, the focus expanded to the use of postnatal stem cells to overcome the encountered problems.
Among the investigated (hematopoietic and mesodermal) stem cell populations are muscle-derived stem cells (MDSCs). MDSCs—not to be confused with satellite cells—are relatively easy to harvest through minimally invasive procedures and possess an inherent ability for regeneration with long-term proliferation, multipotent differentiation, and high self-renewal [77, 81]. Furthermore, MDSCs can be genetically modified to express proteins of interest, for example, growth factors or anti-fibrotic molecules such as decorin [77, 78].
In theory, these cells can either participate in the muscle regeneration by forming new muscle fibers and neurovascular supply, or by delivering growth factors at the site of injury.
However, whether this interesting therapeutic approach could play a beneficial role in the treatment of (acute) muscle injuries is not extensively studied since most available literature focuses on degenerative disorders such as muscular dystrophies.
Current knowledge on the effect of MDSC transplantation for acute muscle injury comes from two (murine) contusion model studies [82, 83]. Direct intramuscular transplantation of MDSCs at day 4 promoted angiogenesis and lead to a significantly higher number and larger diameter of regenerative muscle fibers compared to controls and earlier/later transplantations. However, despite a decrease in the fibrotic area for this group, a considerable amount of fibrosis was still noted [83]. This was attributed to MDSC differentiation into fibroblasts under the influence of local TGF-β1. This prompted a further study combining MDSCs and losartan to decrease fibrosis [82] (for losartan, see “Anti-fibrotic therapy”). When losartan was added to the MDSC treatment, fibrosis decreased and muscle healing was accelerated and further enhanced. These results appear promising. However, the time needed to culture and prepare the stem cells, which depends on the needed amount of cells [83, 84], can be viewed as a possible limitation. Furthermore, extrapolating results from animal studies to humans is problematic. In addition, concerns about clinical application of stem cell transplantation have been raised regarding their potential tumorigenicity. Although this phenomenon has not been observed in practice and may be less likely with postnatal stem cells rather than with embryonic stem cells, it certainly warrants a thorough evaluation of stem cell treatment safety including long-term tumorigenic risk.
An interesting concept that deserves mention is “tissue engineering,” in which stem cells (e.g., MDSCs), a matrix/scaffold and signaling molecules are combined [77, 78, 85]. This is being developed for articular cartilage regeneration and healing of bony defects [77, 78] and could influence future repair strategies for severe muscle injuries/muscle trauma, such as volumetric muscle loss [85].
In conclusion, the (intramuscular) use of stem cells, whether or not modified and/or combined with scaffolds, is an exciting concept that warrants further development and evaluation. Despite promising results, we currently do not advocate its use for (acute) skeletal muscle injuries since its efficacy and safety for human use including long-term outcome have yet to be determined.
19.4.6 Anti-fibrotic Therapy
The formation of connective (scar) tissue occurs during the response that is triggered following muscle injury. However, an aberrant response may lead to the excessive accumulation of scar tissue (i.e., fibrosis). Scar tissue can be a mechanical barrier that may restrict muscle fiber regeneration and reinnervation at the site of injury where the scar is localized [16], hindering a complete recovery of the injured muscle tissue and muscle function [13, 16, 36, 86, 87].
In theory, measures that counteract fibrosis have the potential to enhance muscle healing. TGF-β1 (Transforming Growth Factor-β1) has been identified as a key factor in the development of scar tissue by activating fibrotic cascades [13, 36, 87–89]. As a consequence, anti-fibrotic strategies have mainly targeted the TGF-β1 pathway [13, 87].
TGF-β1 may mediate its pro-fibrotic effect by stimulating gene transcription favoring extracellular matrix deposition through ligand-induced phosphorylation of SMADs, which are intracellular proteins involved in transduction of the extracellular signal in the form of TGF-β1. Following phosphorylation, SMAD2 and SMAD3 (receptor-regulated SMADs, or R-SMADs) bind to SMAD4 (common-mediator SMAD, or co-SMAD) and translocate to the cell nucleus resulting in gene transcription [90]. SMAD7 (as a part of a negative feedback loop) has an inhibitory effect on activation of R-SMADS. Anti-fibrotic strategies may target different parts of this pathway.
There are several anti-fibrotic agents that we will discuss here.
19.4.6.1 Decorin
Decorin, a human proteoglycan, has been reported to block TGF-β1 action on its receptor by binding to TGF-β1 [89]. In a (murine) muscle laceration injury model, a direct injection with decorin significantly decreased the amount of fibrosis and enhanced muscle healing (i.e., higher number and diameter of regenerating muscle fibers, as well as improved muscle function) compared to a control group with a direct injection with saline [86]. However, large quantities of decorin were required in a very small murine muscle, which might limit future use of direct decorin injection in clinical practice.
19.4.6.2 Suramin
Suramin, currently prescribed as an antiparasitic drug, competitively binds the TGF-β1 receptor and thereby has an inhibitory effect on the TGF-β1 pathway [88]. It has been reported to have an antiproliferative effect on fibroblasts in vitro [91]. In murine models, Suramin reduced scar tissue formation and had a positive effect on muscle regeneration [91, 92]. Although no obvious side effects were noted following intramuscular injection in mice, the safety of intramuscular use has not been evaluated in humans.
19.4.6.3 Losartan
Losartan is an angiotensin-II receptor antagonist that is already (FDA) approved for clinical use and is thought to reduce fibrosis by inhibiting activation of R-SMADs through upregulation of SMAD7 [82]. In addition, losartan appears to result in increased local expression of follistatin, which is believed to stimulate angiogenesis and is able to neutralize several members of the TGF-β superfamily [82]. In murine (laceration/contusion) models, oral administration of losartan was found to reduce fibrosis and enhance structural and functional muscle regeneration [93, 94]. Interestingly, a dose equivalent for treating hypertension in humans was tested and found to be effective [94]. In addition, studies focusing on losartan as an addition to interventions such as stem cells [82, 95] or PRP [44] reported similar findings after treatment with losartan. Since losartan is already widely used for hypertension in humans, this could prove an interesting and readily accessible intervention to improve muscle healing. However, studies in human skeletal muscle should be carried out before implementing losartan in the treatment of muscle injuries.
19.4.6.4 Interferon-γ
Interferon-γ also has an inhibitory effect on the TGF-β1 pathway by inducing SMAD7-expression. Intramuscular injection in a murine (laceration) injury model led to a smaller area of fibrosis and improved muscle healing (higher number of muscle fibers and improved muscle function) [96].
These studies support the idea that fibrosis hinders complete recovery of muscle structure and function by demonstrating that muscle regeneration can be enhanced by inhibition of the TGF-β1 pathway in murine models. However, since no clinical trials in humans exist, the logical next step is to evaluate efficacy and safety of the aforementioned anti-fibrotic therapies in humans before they can be used in clinical practice.
19.4.7 Are Intramuscular Injections Safe?
All interventions have a risk of side effects that should be considered. In a 2014 systematic review, possible myotoxic effects of commonly used intramuscular injection preparations were evaluated [97]. This systematic review found evidence for myotoxicity of local anesthetics, nonsteroidal anti-inflammatory drugs, and corticosteroids. For PRP, there was conflicting evidence: two of the included studies showed increased regeneration with less necrosis and granulomatous tissue in muscles injected with PRP compared to controls. Yet, another study reported edema, inflammatory cell infiltrate, necrosis, and fibrosis following PRP injection, which were not observed in the control group.
For emerging approaches, myotoxic or other side effects are often insufficiently known. For example, there is no evidence available on possible myotoxic effects of intramuscular injection of Actovegin® and Traumeel®. In case of paucity of high-level evidence that such new treatment modalities are efficacious in acute muscle injury, clinicians should consider the “Primum non nocere” (“first do no harm”) dogma of Hippocrates before using these treatments in patients.
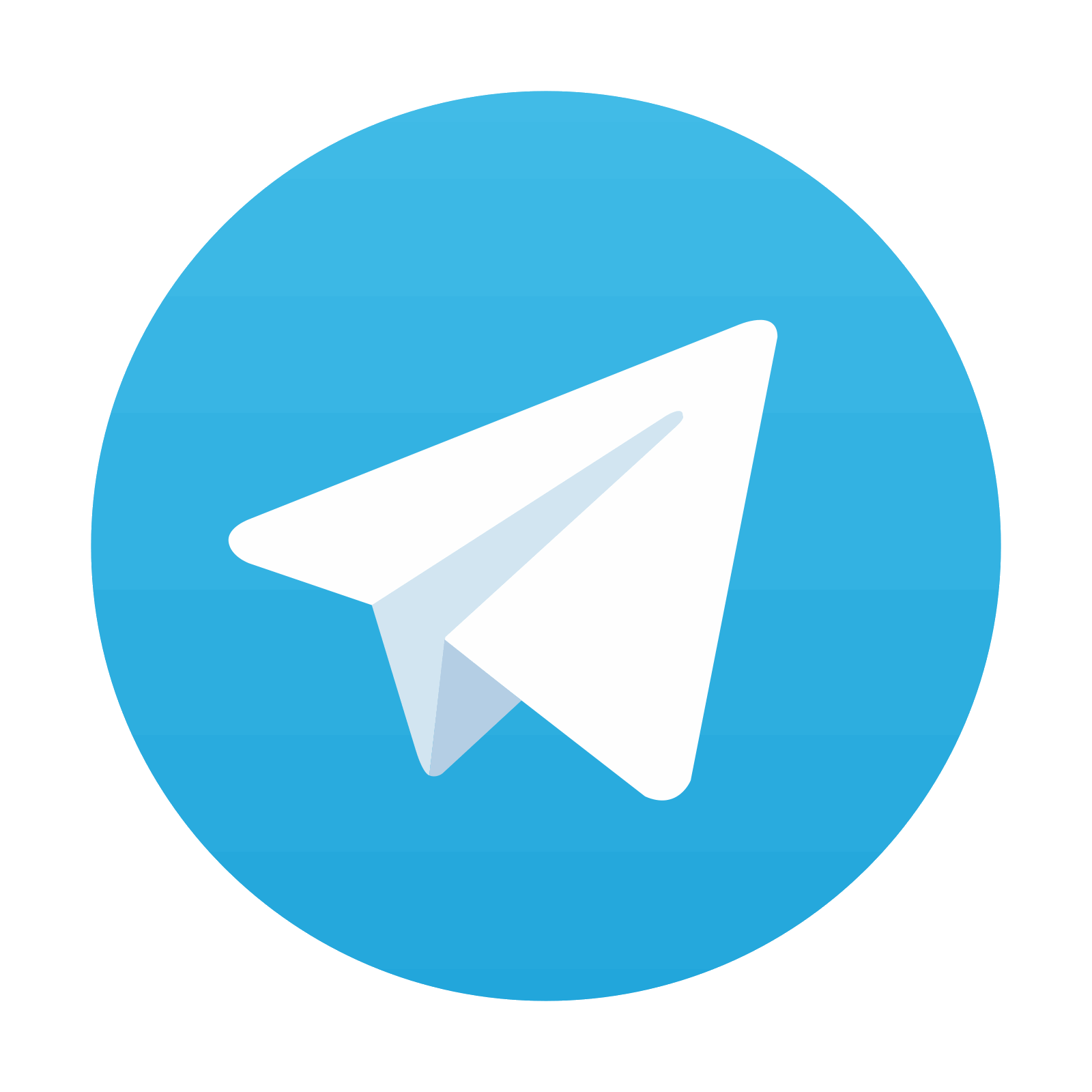
Stay updated, free articles. Join our Telegram channel
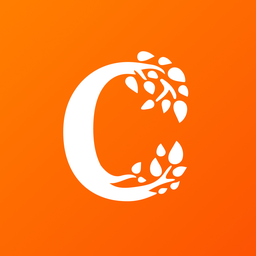
Full access? Get Clinical Tree
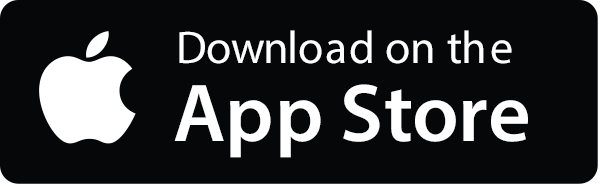
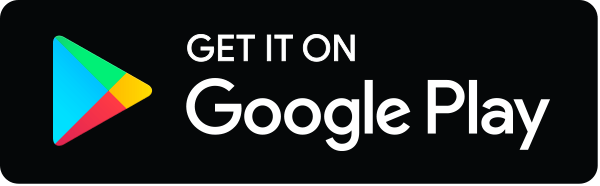