Fig. 2.1
Schematic of sensors, signaling pathways, and responses involved in osteocyte mechanobiology. Much of the current state of knowledge regarding osteocyte mechanobiology is represented in the schematic, although details are lacking in many cases. The detailed signaling mechanisms involved in osteoprogenitor mechanobiology are poorly defined. While osteoprogenitor cells appear to use many of the same sensors (e.g., integrins, ion channels, gap junctions, and primary cilia) and pathways (e.g., ERK1/2 and other MAPKs, Ca2+, and Wnt) as osteocytes, their responses to distinct mechanical stimuli often differ and are not as well defined. Used with permission from Chen J-H, Liu C, You L, Simmons CA. Boning up on Wolff’s Law: mechanical regulation of the cells that make and maintain bone. J Biomech. 2010;43:108–18
Protein Kinase Activation
Mechanical forces are particularly effective at activating mitogen-activated protein kinase (MAPK) cascades in nearly all types of bone cells evaluated. MAPKs are serine/threonine protein kinases necessary for bone cell differentiation, proliferation, and survival. In endothelial cells within bone, mechanical factors activate not only extracellular signal-related kinases 1 and 2 (ERK1/2) but also p38, beta-cell myeloid kinase 1 (BMK-1), and c-Jun terminal kinases (JNK). Mechanical activation of ERK1/2 has been shown to be critical for certain strain responses in bone stromal and osteoblastic cells [3]. ERK1/2 causes downregulation of receptor activator of nuclear factor kappa-B ligand (RANKL) and upregulation of endothelial nitric oxide synthase (eNOS) protein after bone cells are exposed to strain. Activation of these pathways leads to decreased bone resorption and increased bone formation.
Activation of ERK1/2 is also necessary for transforming growth factor-β (TGFβ)-induced osteogenic differentiation of mesenchymal stem cells (MSCs) into preosteoblasts and osteoblasts. ERK1/2 activation in bone cells also occurs during mechanical stimulation of voltage-sensitive calcium channels (VSCCs). Activation of ERK1/2 in osteoblastic cells by fluid shear requires Ca2+ influx through VSCCs and is ATP dependent. Osteocyte-like MLO-Y4 cells require association of the α2δ1 auxiliary subunit with VSCCs in order for mechanical signals to activate ERK1/2.
Protein kinase B (Akt) is a serine/threonine kinase involved in a broad range of cellular functions that is activated by a variety of growth factors, cytokines, and mechanical signals [4]. Mechanical signals that activate Akt lead to increased β-catenin activity, which causes inhibition of MSC adipogenesis, thereby causing differentiation of bone marrow MSCs toward the osteoblast lineage. Exposure to mechanical strain leading to Akt activation results in increased focal adhesion assembly in bone cells. Skeletal loading resulting in increased bone strength is influenced by refractory periods that allow regeneration of enzymes, recycling of molecules to the cell surface, and the numbers and arrangement of cytoskeletal platforms initiating the signaling cascade.
Focal adhesion kinase (FAK) is a non-receptor cytoplasmic protein tyrosine kinase (PTK) that is found in higher concentrations near focal adhesions between cells. This kinase plays an important role in signaling events involving growth factors, extracellular matrix (ECM) molecules, and stress signals [5]. FAK is found in proximity to signaling proteins including sarcoma gene (Src) family PTKs, phosphatidylinositol-3-kinases (PI3K), and paxillin. Interactions with these associated signaling proteins enable FAK to form a functional network of integrin-stimulated signaling pathways that result in activation of downstream targets, including MAPK pathways. Activation of FAK leads to autophosphorylation of FAK tyrosine 397, which leads to interactions with Src-family proteins and other molecules containing Src homology 2 (SH2) domains. Phosphorylation of FAK leads to MAPK activation by interacting with chicken sarcoma gene (c-Src), growth factor receptor-bound protein 2 (Grb2), and rat sarcoma gene (Ras), explaining why most biophysical stimuli in cell culture cause activation of MAPK pathways. Oscillatory fluid flow (OFF) has been demonstrated to cause sustained association of Src and FAK with αvβ3 integrin. Stimulation of FAK caused by this integrin association upregulates PI3K activity and modulates downstream ERK and Akt/mammalian target of rapamycin (mTOR)/p70S6K pathways, leading to increased osteoblast proliferation. FAK has also been shown to contribute to OFF-induced stimulation of osteopontin (OPN) and cyclooxygenase-2 (COX-2) expression in osteoblasts. This effect is critical for fluid shear stress-mediated increased expression of osteocalcin (OCN), core-binding factor subunit α1 (Runx2), and Osterix (Osx), demonstrating that FAK is important for osteoblast differentiation and bone formation.
β-Catenin
β-Catenin is critical for bone formation by osteoblasts, but plays other roles in bone biology. A mutation in the Wingless (Wnt) co-receptor lipid-related protein receptor-5/6 (LRP5/6) that causes constitutive activation of Wnt stimulates bone formation leading to a high bone mass phenotype known as sclerosteosis, and a dominant negative mutation of the same LRP5/6 receptor results in a low bone mass phenotype known as osteoporosis-pseudoglioma syndrome. Multiple studies have demonstrated the role of β-catenin in osteoblasts and osteocytes. β-Catenin regulates both bone formation and resorption [6, 7]. Skeletal loading has been shown to regulate levels of β-catenin in bone cells in animals and humans.
While the LRP5/6 receptor may function as a mechanoreceptor in osteoblasts, mechanical strain has been shown to control MSC differentiation via non-LRP5/6 regulation of β-catenin. Two percent mechanical strain at 10 cycles/min and continuous fluid flow at 8 dyn/cm2 and OFF at 10 dyn/cm2 have each been shown to stimulate β-catenin activity in osteoblasts in spite of blocked LRP5/6 receptors. Low-intensity vibration at <10με and 90 cycles per second stimulates β-catenin and suppresses fat cell differentiation, indicating that both high- and low-magnitude mechanical stimuli alter MSC fate through β-catenin. Focal adhesion-based connections with substrate mediate glycogen synthase kinase-3β (GSK3β) inhibition after loading of MSCs and osteoblasts, resulting in increased β-catenin due to reduced proteosomal degradation. Proximal signaling results in mechanical activation of mTORC2, leading to phosphorylation of Akt serine 473, leading to Akt-dependent inhibition of GSK3β (Fig. 2.2). If mTORC2 functions as a mechanical target, there may be interactions between the cytoskeleton and metabolic responses to exercise since mTORC2 responds to insulin signaling.


Fig. 2.2
Force-induced activation of β-catenin. Application of mechanical force to cells induces focal adhesion-dependent activation of mTORC2. mTORC2 then activates Akt, which inactivates GSK3β via phosphorylation. Inhibition of GSK3β leads to multiple downstream events, including preservation and nuclear translocation of β-catenin, as well as prolonging the nuclear residence of NFATc1. Used with permission from Thompson WR, Rubin CT, Rubin J. Mechanical regulation of signaling pathways in bone. Gene. 2012;503:179–93
GTPases and G-Protein-Coupled Receptors
Guanosine triphosphatases (GTPases) are a large family of enzymes that bind to and hydrolyze GTP. These enzymes function as switches regulating a wide variety of physiological processes. Mechanical stimuli activate heterotrimeric GTPases via G-protein-coupled receptors, resulting in increased intracellular calcium ([Ca2+]i), cyclic AMP (cAMP), and cyclic GMP (cGMP) (Fig. 2.3). Nitric oxide (NO) is produced by protein kinase G (PKG) after mechanical shear stress in osteoblasts [8]. PKGII was shown to be necessary for Src upregulation by phosphorylating Src homology 2 domain-containing tyrosine phosphatase-1 (SHP-1). Subsequent fluid shear stress led to recruitment of PKGII, Src, SHP-1, and SHP-2 to a β3-integrin-containing mechanosome.


Fig. 2.3
Mechanical activation of Rho via focal adhesions. Forces transmitted through focal adhesions are known to activate signaling cascades, possibly though force-induced conformational change. Involved signaling pathways include focal adhesion kinase (FAK), ERK1/2, and Src. ERK and Src can initiate Rho signaling via activation of GEFs (here shown as GEF-H1 and Larg). GDP bound RhoA is critical for assembly of new focal adhesions and stress fiber polymerization. Used with permission from Thompson WR, Rubin CT, Rubin J. Mechanical regulation of signaling pathways in bone. Gene. 2012;503:179–93
Estrogen Receptors
Estrogen deficiency after menopause is a major cause of osteoporosis, and estrogen receptors (ERs) play a key role in postmenopausal bone loss. ERα mediates bone formation in response to load-bearing in vivo and regulates how osteoblasts and osteocytes respond to mechanical stimulation [9]. ER also modulates mechanically activated signaling pathways. ERα knockout (KO) mice were shown to have reduced response to tibial loading, with severely reduced and delayed transcriptional response and a broad range of effects compared to wild-type littermates. Three hours following a brief loading regimen, wild-type mouse tibiae showed altered transcription of 642 genes, while only 26 genes were modified in ERα KO tibiae. For example, sclerostin gene (SOST) expression was significantly reduced after loading in wild-type mice, but unchanged in loaded tibiae of ERα KO mice.
Bone cells respond to mechanical stimuli via ERα through both genomic and nongenomic actions. ERα nongenomic actions depend on direct interaction with insulin-like growth factor-1 receptor (IGF-1R), leading to sensitization of IGF-1R and upregulation of early strain-regulated genes including COX-2. β1-Integrin expression, which is important for load-induced bone formation, is also upregulated by ERα. These interactions help explain why estrogen may help upregulate osteogenic target genes in response to mechanical stimuli.
Calcium Signaling
A rapid increase in [Ca2+]i is the earliest detectable response in mechanically activated bone cells [10]. Calcium channels in the plasma membrane and intracellular organelles help regulate [Ca2+]i. [Ca2+]i concentrations are tightly regulated to maintain a very low level of free [Ca2+]i, making [Ca2+]i an excellent second messenger system. [Ca2+]i serves as an initial signal in bone cell proliferation, mitosis, differentiation, and motility. [Ca2+]i mobilization is initiated by cell membrane strain, pressure, fluid flow, and osmotic swelling. The frequency of [Ca2+]i spiking is more important in bone cell response to loading than the magnitude of Ca2+ spikes, and a rest period between loading enhances Ca2+ response in osteoblastic cells.
Changes in [Ca2+]i are linked to several mechanically regulated signaling cascades, including inositol-3-phosphate (IP3), adenosine triphosphate (ATP), and NO. [Ca2+]i mobilization stimulates downstream signaling through protein kinase A (PKA), MAPK, and c-Fos. Prostaglandin E2 (PGE2) release plays an important role in mechanical bone formation, but is activated by a Ca2+-independent mechanism. Other studies show that PGE2 release is dependent on Ca2+ entry through the L-type VSCC, followed by release of ATP.
Influence of Mechanical Loading on Mesenchymal Stem Cell Differentiation
Mechanical strain applied to mesenchymal stem cells causes these cells to differentiate toward osteoblasts and suppresses differentiation toward adipocytes [11]. Bone formation occurs during embryonic growth and development and during postnatal bone modeling, remodeling, and repair. In order for osteoblasts to continue to fulfill their role, the skeleton or other tissues must contain a sufficiently large reservoir of osteoprogenitor cells to generate new osteoblasts from birth until death. Osteoprogenitor cells maintain their ability to differentiate from a less differentiated precursor state, or to transdifferentiate from other differentiated cell types, into osteoblast-like cells that produce a variety of proteins characterizing osteoblasts, such as Runx2, alkaline phosphatase (ALP), ON, and OCN, and synthesize bone matrix. Because osteoprogenitor cells have not yet been identified to express specific protein markers, they are difficult to identify, and the precise anatomical location of their niche remains unknown. Osteoprogenitor subpopulations have been identified in bone marrow, but also in cardiovascular tissues, including the aortic heart valve, vascular smooth muscle, and capillary beds. Mechanical signals are also thought to regulate the function and differentiation of osteoprogenitor cells in tissues other than the bone.
Substrate Deformation Effects on Osteoprogenitor Cells
Mechanical strain induces differentiation of osteogenic precursors and suppresses differentiation of osteoprogenitor cells into adipocytes. After being stretched for over 24 h, mRNA transcripts for ALP, Runx2, BMP2, BMP4, and collagen type I are significantly increased in osteogenic precursors. After cyclic stretching for 1–2 weeks, osteogenic precursor OCN and Runx2 protein levels increase, and mineralization of deposited matrix occurs. The extent of the osteogenic response depends on the size of the strain detected and mediated through FosB activation [12]. How osteoprogenitor cells respond to mechanical strain depends on their stage of differentiation. Mechanical strain at less differentiated stages leads to increased Runx2 and type I collagen mRNA expression, decreased proliferation of osteoprogenitors, and increased apoptosis, compared to exposure at more differentiated stages.
Stretching the extracellular matrix applies force to cell focal adhesions to the matrix, which results in increased FAK activity, activating downstream MAPK signaling pathways. Mechanical strain induces rapid phosphorylation of MAPK. Although mechanical strain increases MAPK activity, and inhibition of MAPK in cell culture decreases mineralization, inhibition of these pathways has little to no effect on strain-induced osteogenic differentiation in osteoprogenitor cells found in blood vessels. These findings suggest that mechanical strain also activates other signaling pathways to stimulate osteogenic differentiation.
Certain calcium channels may be activated by mechanical strain. Blockage of these calcium channels pharmacologically, or culture of osteoprogenitor cells in calcium-free media, leads to decreased strain-induced ALP upregulation. The Wnt signaling pathway may be stimulated mechanically to promote bone formation. Mechanical strain upregulates Wnt 10B and frizzled-2 receptor mRNA, which leads to increased phosphorylated β-catenin nuclear translocation in osteoprogenitor cells.
Fluid Flow Effects on Osteoprogenitor Cells
Fluid flow in the intramedullary space and Haversian canals occurs in response to mechanical strain, suggesting that fluid flow stimulates osteoprogenitor cells in these compartments also. Osteoprogenitor cells appear to be more responsive to shear stress than matrix deformation when these are both applied at the physiological ranges seen in the bone.
Although most studies have reported that fluid flow promotes osteogenic differentiation, some studies have not. In parallel plate flow chamber studies, flow-induced shear stress stimulated mRNA expression of some preosteoblast markers, but had no effect or inhibited other preosteoblast markers such as ALP, Runx2, or osteocalcin. These findings were attributed to differences in cell substrate surface chemistry and different flow patterns employed. ECM proteins and substrate materials are known to influence osteogenic differentiation, but studies have not thoroughly explored the effect of matrix proteins in combination with mechanical strain. One study demonstrated that the calcium phosphate coating on glass slides was associated with less flow-stimulated OPN and bone sialoprotein mRNA synthesis, suggesting that matrix properties affect osteoprogenitor cell mechanosensation. Osteopontin mRNA expression and ERK1/2 phosphorylation are variable, depending on whether the flow pattern is continuous, intermittent, or oscillatory and the magnitude of shear stress and flow frequency. Variable osteoprogenitor response to flow pattern may also explain some of the discrepancies seen between different studies.
The effect of flow on osteoprogenitor cells has also been evaluated in three-dimensional cell culture systems. In this case, cells are seeded into hydrogels or scaffolds and then cultured during spinning or flow perfusion. Similar to what is found in 2D systems, fluid flow stimulates preosteoblast differentiation in 3D systems. Fluid-induced preosteoblast differentiation in 3D systems also depends on substrate material. Even though 3D systems better reflect the in vivo cellular environment, the effects of shear stress and mass transport are difficult to separate. In addition, variability in pore size and interconnectivity of the scaffolds make it difficult to quantitate shear stress experienced by the cells.
Primary cilia, gap junctions, and the cytoskeleton are important in osteoprogenitor cell mechanosensing of fluid flow [13]. Primary cilia are nonmotile and project from the cell membrane into the extracellular space and move with the flow. Mechanosensing through primary cilia in osteoblast precursors is independent of calcium flux and stretch-activated channels and required for flow-induced stimulation of osteoblast differentiation, as pharmacological removal of primary cilia prevents flow-induced stimulation of OPN mRNA and PGE2 secretion. Pharmacological inhibition of gap junctions in osteoprogenitor cells caused similar results.
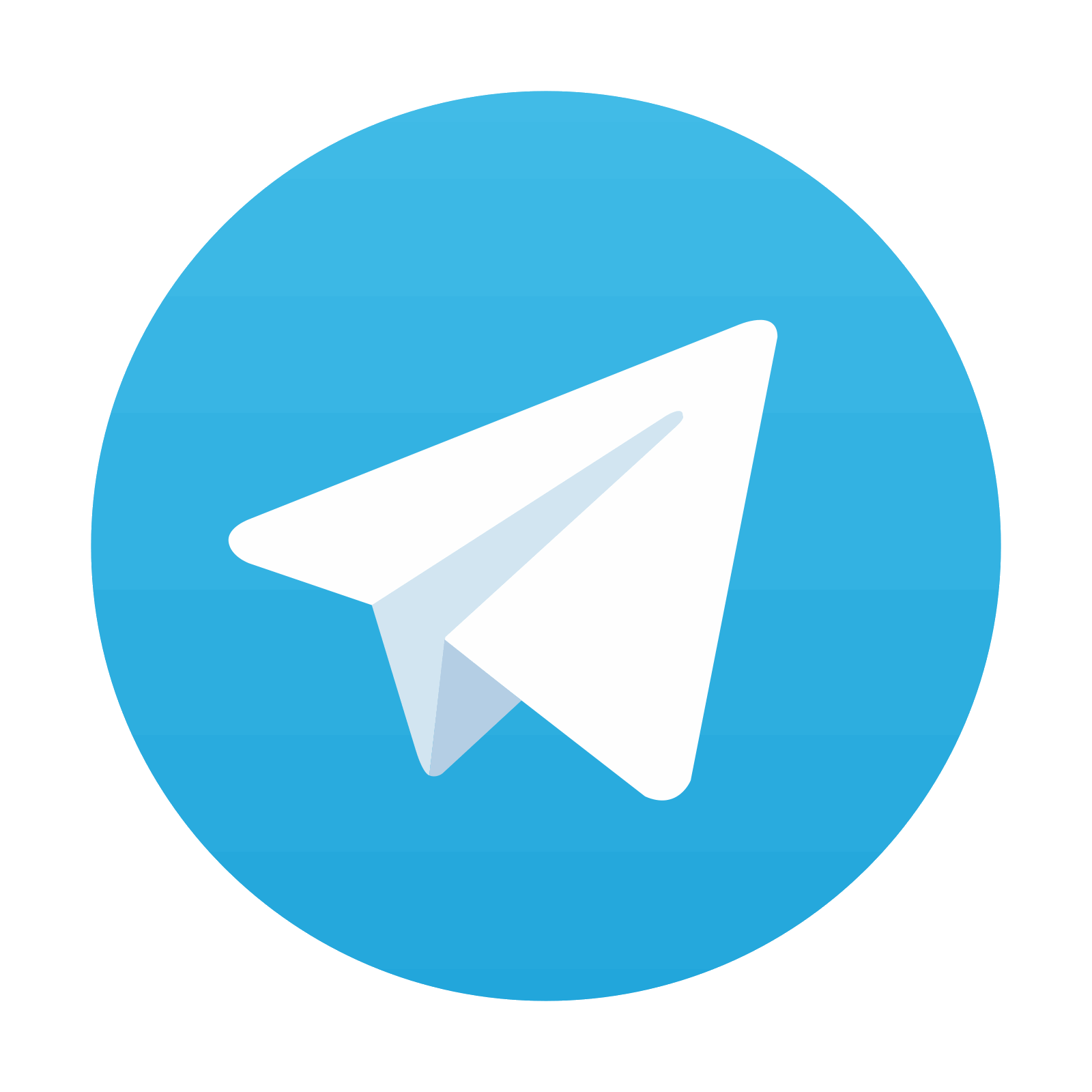
Stay updated, free articles. Join our Telegram channel
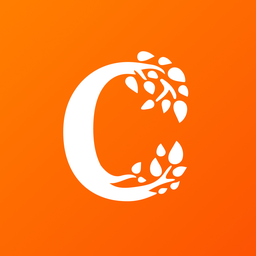
Full access? Get Clinical Tree
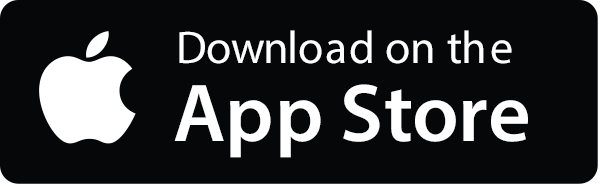
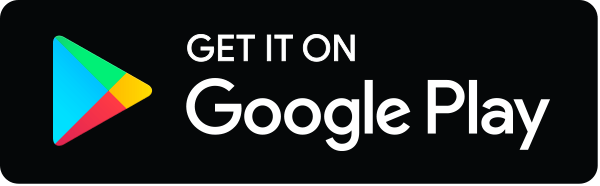