Fig. 5.1
Humoral mediators and associated pathways drive anabolic and catabolic responses in the skeletal muscle (Costamagna et al. [40])
5.3 Muscle Injury and Immune Cells
Even though molecular regulation of myogenesis and skeletal muscle regeneration are almost indistinguishable, the immune response is observed solely in harmed muscle. Immune cells are of myeloid origin and amount to high numbers (105/mm3) in damaged muscle site. Immune cells actively secrete numerous cytokines and growth factors in order to modulate own inflammatory activity, and they affect the viability and transcriptional activities of regenerating muscle cells [190]. Most of the biological effects observed in the immune system are mediated by cytokines divided into two groups: one represented by pro-inflammatory activity and second suited by anti-inflammatory action. Nowadays, there is a strong belief that the balance between pro- and anti-inflammatory cytokines is essential for accurate immune response to injury. Although expression of myogenic genes occurs in the absence of myeloid cells, the latter can control the extent and timing of expression. It is obvious that some cytokines endorse muscle satellite cell proliferation in vitro [29, 109].
T lymphocytes embody major source of cytokines with CD4-expressing T lymphocytes (CD4+) also known as helper cells, the most useful cytokine producers. CD4+ T lymphocytes could be further subdivided into Th1 and Th2, while the cytokines they secrete are termed Th1-type (pro-inflammatory such as interferon-γ, TNF-α, interleukin 1β) and Th2-type (anti-inflammatory such as interleukins 3, 5, 10, and 13), respectively. Inflammatory lesion in skeletal muscle begins the fast infiltration with Ly6C+/F4/80− neutrophils during acute phase of inflammation (the second hour) peaking between 6 and 24 h postinjury. This phase ends up with phagocytic CD68+ macrophages of M1 phenotype that rise in number between 24 and 48 h postinjury. M1 macrophages decline sharply 2 days postinjury, and this decline heralds the infiltration of non-phagocytic CD206-expressing macrophages of M2 phenotype (M2a, M2b, M2c) [170, 191]. The induction of MRF in skeletal muscle progenitors is in striking coincidence with the release of inflammatory and vascular mediators by immune cells. First, the degree of inflammatory reaction affects regenerating muscle, with greater injury observed in wide-ranging infiltration by neutrophils and M1 macrophages. Free radicals (oxygen, nitrogen, and chlorate species) released by neutrophils and M1 macrophages lead to plasma membrane degradation (lipid peroxidation) and an extracellular debris for phagocytosis [27]. Thus, reductions in phagocyte-mediated damage facilitate and accelerate muscle regeneration [220].
One must bear in mind that innate immune response begins with TNF-α- and interferon-γ-driven activation of macrophages. These cells acquire M1 pro-inflammatory phenotype with vast generation of nitric oxide (NO) by inducible form of nitric oxide synthase (iNOS). By the way, other destructive nitrogen species such as peroxynitrite could come out [201]. Importantly, M1 macrophages express CD68, the receptor for oxidized LDLs which in turn stimulates phagocytosis and secretion of pro-inflammatory cytokines [153].
TNF-α plays a critical role in inflammation as this is the first cytokine released by neutrophils and M1 macrophages to trigger production of other Th1 cytokines. Besides, TNF-α was frequently reported to encourage skeletal muscle regeneration at early stage following acute injury as TNF-α null mutants and TNF-α receptor mutants had lower expression levels of MyoD and MEF-2 than wild-type controls [35, 204]. In differentiating C2C12 myotubes, TNF-α administration augmented cell growth [103], whereas it inhibited MyHC IIa protein expression in differentiated myotubes [145, 146]. Apparently, muscle cell fusion is inhibited, whereas myoblasts proliferation is stimulated by TNF-α that might trigger network of complex intracellular signaling pathways engaging NF-κB, signal transducer and activator of transcription (STAT-1α) and other transcription factors [120, 145, 146]. NF-κB activation by alternative pathway plays important function in skeletal muscle, as it promotes the expression and stability of cyclin D1 leading to increased cell proliferation and inhibition of differentiation [73]. Another explanation of NF-κB-dependent skeletal muscle growth retardation comes from the observation that MyoD and MyoD mRNA template are NF-κB targets for degradation [91]. Finally, NF-κB was shown to bind skeletal muscle transcriptional repressor, namely, YY1 which depresses activation of muscle-specific genes [203]. Thus, the exact role of TNF-α in muscle growth and regeneration varies on the degree of muscle damage, particular phase (transition from early to terminal differentiation), and cellular target such as NF-κB [218]. Although TNF-α levels peak at 24 h postinjury, this cytokine levels remain elevated up to 2 weeks following acute muscle injury which advocates principal role of TNF-α played in skeletal muscle repair [204]. It also points to TNF-α targets other than NF-κB muscle regulatory pathways, possibly p38 MAP kinase signaling [221]. Blocking the p38 kinase pathway resulted in diminished myogenesis and increased NF-κB activity in C2C12 myotubes [90]. TNF-α-dependent effects on muscle growth/regeneration are also associated with its chemoattractant attribute to stimulate directional migration of myoblasts and satellite cells [195]. Presumably, when TNF-α is released from polymorphonuclear cells (PMCs) and M1 macrophages at the site of injury, it is chemical attractant for muscle stem and progenitor cells, by sensing this cytokine peel direction where the cell damage is to be compensated. Chemoattraction can be inhibited by deletion of TNF-α with specific antibodies or by the suppression of M1 macrophages [106, 115]. Elevated muscle protein loss is another important product of TNF-α activity regardless of circumstances. It is now well established that this effect of TNF-α is associated with induction of FBXO32/Atrogin1 gene and its expression product atrogin-1 protein [24, 145, 146] mediated by p38 MAPK [103]. Apparently, TNF-α plays two opposite roles in muscle repair, one related to degenerative phase where it stimulates proliferation and migration of muscle stem and progenitor cells and the second where it holds back regenerative phase by impaired muscle cell fusion and accelerated protein decay [68, 145, 146].
Immune, type II, or γ-interferon (IFN-γ) is secreted by thymus-derived (T) cells under certain conditions of activation and by natural killer (NK) cells. IFN-γ controls several aspects of the immune response, comprising stimulation of bactericidal activity of phagocytes, stimulation of antigen presentation through class I and class II major histocompatibility complex (MHC) molecules, or by orchestration of leukocyte-endothelium interactions linked to cell proliferation and apoptosis [25].
IFN-γ is also another pro-inflammatory cytokine of Th1 series widely known from its pleiotropic activity taking in muscle growth and repair [36, 61]. It is worth noting, IFN-γ cooperates with TNF-α in many aspects of initial adaptation to skeletal muscle injury [10, 145, 146, 194, 207, 208]. Furthermore, IFN-γ and TNF-α relations are also evident during muscle cell differentiation when muscle fibers grow up and develop [145, 146, 168]. While early stages of skeletal myogenesis are apparently stimulated by both cytokines (augmented proliferation), later phases are markedly hampered, with noticed ATP-dependent proteolysis associated with E3 ubiquitin ligases atrogin-1/MAFbx and MuRF1 [145, 146]. The latter muscle-specific RING-finger protein 1 ligase and MAFbx are indicated as important IFN-γ and TNF-α targets in cancer cachexia [1]. Transcriptome analysis of differentiating C2C12 muscle cells revealed that IFN-γ affects the expression of several genes. The cytokine promoted cytokine/growth factor expression, cell proliferation, and migration but impaired muscle cell differentiation [72]. Presumably, the implementation of such a variety of effects by a single cytokine is achieved by complex patterns of skeletal muscle cell-specific gene regulation with IFN-γ response regulated by interaction with responses to other cytokines including TNF-α.
IFN-γ blocks the secretion of Th2 cytokines bulk which inhibits the inflammatory response. Thus, IFN-γ probably upholds inflammatory response of injured muscle in order to postpone tissue repair unless the population of muscle progenitor cells is sufficient for muscle regeneration. Th2 cytokines represented by IL-4, IL-10, and IL-13 stimulate M2 macrophages to take over M1 macrophages [69]. There are several subcategories of M2 macrophages indicated by small letters (M2a, M2b, M2c) (Fig. 5.2).


Fig. 5.2
Secreted molecules and paracrine effects from resident and circulating cells involved in skeletal muscle inflammation (Costamagna et al. [40])
5.4 Skeletal Muscle as an Important Source of IL-6
Myokines, a term used to describe a group of cytokines produced by skeletal muscle during and following exercise, point to a brand new role played by this organ [138]. Except MGF, remaining myokines are identical to previously described and identified leukocytes (IL-6, IL-1, interleukin 1 receptor antagonist (IL-1ra), IL-8, macrophage inflammatory protein α and β (MIP-1α and MIP-1β), TNF-α). Nowadays, it is widely recognized that strenuous prolonged exercise, e.g., marathon run, causes huge increase in the concentration of several pro- and anti-inflammatory cytokines in the peripheral blood [51, 52, 138]. Previously, it was well established that physical exercise stimulates the leukocytosis (increased number of leukocytes in peripheral blood). However, the origin of myokines is different from immune cells as both transcripts and proteins concentrate in skeletal muscles [66, 86, 176, 179]. The most prominent among myokines is IL-6 for the reason that following exercise this cytokine basal plasma levels may increase up to 100-fold [58, 132, 135, 140–142]. The peak IL-6 plasma concentration is observed at the end of the exercise or soon after [56, 57, 58, 132]. For a long time, IL-6 has been considered as classical inflammatory cytokine suggesting that muscle fiber injuries promote almost exponential raise of the cytokine plasma level during a bout of heavy exercise. This assumption has no evidence as increase in IL-6 is observed in both non-damaging (concentric) [211] and damaging (eccentric) skeletal muscle contractions [107]. What is the role of IL-6 and why this myokine is that much upregulated in exercised skeletal muscle? Since IL-6 release by skeletal muscle is proportional to the muscle mass, exercise intensity, and duration (twofold increase in IL-6 level was observed after 6 min, tenfold after 2 h, and 100-fold after 6 h of running), there is presumption that this myokine is of paramount importance for homeostasis in contracting muscles, as resting muscles exposed to identical hormonal milieu do not release IL-6 [78]. Fluorescence-activated cell sorting (FACS) of peripheral monocytes isolated from blood samples of cycling or running athletes did not demonstrate any significant changes in IL-6 even during prolonged exercise [173–175]. These and other experiments show that contracting skeletal muscle is the major source of IL-6 in the peripheral blood in response to exercise and that muscle fibers but not myoblasts, endothelial cells, fibroblasts, or smooth muscle cells account for the systemic increase of plasma IL-6 [77, 86, 108, 143]. Interestingly, exercise-induced increase in IL-6 release from the exercised legs (based on arteriovenous difference) could be blocked completely by the supplementation of vitamins C and E for 4 weeks prior to the challenge [56, 57]. It appears that IL-6 gene activation is redox mediated in skeletal muscles following exercise as antioxidants abolish this effect. Ablation of redox stimulus was also observed in differentiating myoblasts following vitamin C preincubation [131]. Given that IL-6 derived from skeletal muscle is the normal physiologic response to exercise, one may ask about its role and significance. IL-6 appears to be involved in glucose homeostasis [181, 66], and IL-6 response to exercise is dependent on pre-exercise skeletal muscle glycogen content [176]. Moreover, carbohydrate supplementation attenuates the increase of plasma IL-6 of skeletal muscle origin [174, 175]. In addition, IL-6 infusion is accompanied by extensive lipolysis and accelerated fat oxidation pointing to its metabolic rather than immunologic function [199]. Nowadays, the consistent findings indicating IL-6 secretion from exercised muscles in the absence of noticeable inflammatory markers designate this cytokine as a master switch that stimulates utilization of neutral fat when carbohydrates are in shortage. With respect to IL-6, skeletal muscle is prompted to transcriptional, translational, and secretory activities in reaction to rise in sarcoplasmic Ca2+ level during repetitive contractions. Importantly, the effect is calcineurin/nuclear factor of activated T-cell (NFAT) dependent (inhibited by cyclosporin A and potentiated by ionomycin). Sustained intracellular Ca2+ is a well-known calcineurin activator which is calcium- and calmodulin-dependent serine/threonine protein phosphatase upstream to NFAT [62]. Furthermore, IL-6 gene expression at transcriptional and translational levels is controlled by calcineurin/NFAT in cultured muscle cells [86]. NFAT may be rephosphorylated by NFAT kinases like glycogen synthase kinase (GSK-3β) which makes NFAT inactive and withdrawn from the nucleus [54]. We observed critical role played by GSK-3β in myogenesis, as both insulin and metabolic inhibitors of this kinase led to ameliorated muscle fiber formation [104]. Strict pro-inflammatory TNF-α is repressed in the same circumstances (exercised muscle) demonstrating that IL-6 and TNF-α are regulated differently in skeletal muscle cells by altered Ca2+ levels [84]. In addition, other possible signaling pathways explain IL-6 increase in exercised skeletal muscle. Abundantly expressed neuronal NO synthase isoform (nNOS) points toward NO production within contracting skeletal muscles with cGMP and/or nitrosative mechanisms leading to IL-6 production [93, 94, 156, 167]. Bulk of evidence collected from the experiments with NO donors showed increase in IL-6 mRNA content and IL-6 release from skeletal muscles challenged with NO, whereas opposite response was noted upon the use of nNOS inhibitors [178]. Nuclear factor kappa light chain enhancer of activated B cells (NF-κB) is another candidate as it is redox-sensitive transcription factor, while trained skeletal muscle is a rich source of reactive oxygen species (ROS) [167]. Antioxidants were mentioned as IL-6 repressors [189, 200]; thus it is very likely that ROS formation in skeletal muscle following exercise causes IL-6 release in a NF-κB-dependent manner. In order to position NF-κB in the redox-dependent cell signaling, nonsteroidal anti-inflammatory drugs (NSAIDs) were used as NF-κB inhibitors [88]. Actually, indomethacin diminishes the exercise-induced increase of IL-6 further supporting the notion of causal relationship between skeletal muscle contractions, NF-κB and IL-6 synthesis [155]. Moreover, basal IL-6 plasma concentrations are highly correlated with the skeletal muscle training, being lower in trained vs. non-trained individuals [32, 137]. Despite the marked rise in IL-6 mRNA during exercise, the long-term training (1 h, 5 times a week for 10 weeks) led to almost ten times lower IL-6 mRNA content in exercised skeletal muscles [56, 57]. Concomitantly IL-6R mRNA content was almost doubled in trained skeletal muscle [85]. It reveals the nature of feedback mechanism of IL-6 myokine; while plasma IL-6 is apparently downregulated by training, the muscular expression of the IL-6R is upregulated at the same time to augment IL-6 sensing by muscle fibers. The last but probably not the least, signaling pathway activated in skeletal muscle during contraction is p38 MAPK known as stress-activated protein kinase [21]. In fact, fatigued muscle with reduced glycogen content has been found to boost the p38 MAPK activity and its nuclear translocation [33]. Moreover, nuclear p38 MAPK phosphorylation through MAPK kinase (MKK-3 and MKK-6) is known to activate IL-6 gene since inhibition of p38 MAPK results in loss of the IL-6 mRNA transcriptional control [33]. For years, p38 MAPK was shown to be essential for muscle fiber formation in myogenesis; thus its additional control of IL-6 gene brings the role of the kinase updated [41, 90, 210]. It is very likely that the downstream targets of p38 MAPK, ATF-2, and Elk1 may participate in regulating the expression of IL-6 gene, owing to the fact that ATF-2 is a subunit of the AP-1 heterodimer (Jun:ATF) [198], while Elk1 is a member of the Ets superfamily of transcription factors [217].
5.5 Cytokines Negatively Targeting Skeletal Muscle
Chronic systemic inflammation (CSI) is a cause of cardiometabolic syndrome (CMS) associated with higher risk of cardiovascular disease, type 2 diabetes, and muscle cachexia (muscle wasting) [44, 53, 141, 142, 149, 157, 192, 209]. Two- to fourfold rise in plasma concentrations of pro- and anti-inflammatory cytokines and acute-phase proteins are regarded as a common attribute of CSI [28]. Regular exercise prevents CMS almost certainly through the elevated levels of anti-inflammatory cytokines [IL-1ra, soluble TNF-α receptors (sTNF-R), and IL-10] which in turn override inflammatory response [134, 136, 139]. Throughout CSI several pro-inflammatory cytokines are elevated in blood plasma, TNF-α, IL-1β, and IL-6, even though TNF-α plays the central role in insulin desensitization of skeletal muscle [147, 148, 196]. Insulin resistance results in marked decline of its anabolic effect and accelerated loss of skeletal muscle mass typical for type 2 diabetes [147, 148]. A bulk of evidence confirms that TNF-α-induced insulin resistance is associated with NF-κB and STAT-1α activation and inhibition of PI3-K/Akt signaling pathway [26, 145, 146]. Thus, TNF-α represents the causal factor of insulin resistance [147]. It appears that the role of IL-6 in muscle decays as pro- or anti-inflammatory cytokine is less clear as indicated by conflicting data [141, 142, 149, 172, 177]. Observations indicate that IL-6-deficient animals (knockout mice) have higher levels of circulating TNF-α [119], while IL-6 inhibits TNF-α formation [55, 110]. Additionally, IL-6 stimulates formation of anti-inflammatory cytokines IL-1ra and IL-10 [177]. IL-1ra is of particular importance as it weakens inflammatory response being the efficient blocker of IL-1α and IL-1β binding to cognate receptors [45]. Once it is established that IL-6 has anti-inflammatory rather than pro-inflammatory characteristics, the rationale for protective role of physical activity against muscle wasting is to be revealed. Nonetheless, there is no direct relationship between exercise and reduced CSI although several studies have reported improved status of patients with CMS [67, 180]. To decipher the physiological role of IL-6, further studies are needed, as IL-6 levels are augmented both in patients with metabolic disease featured by insulin resistance and hyperlipidemia [37, 129] and after strenuous exercise which improves insulin-stimulated glucose uptake and plasma lipid profile [212]. This actually contradicting observations might be explained by simultaneous alterations in TNF-α concentration as increased TNF-α and decreased IL-6 transcription lead to higher incidence of diabetes [89]; however, the exact etiology of the CMS has at least few scenarios including elevated IL-6 as a systemic feedback mechanism to adipocyte-secreted TNF-α or alternative route to sensitize skeletal muscle to insulin or perhaps it results from “IL-6 resistance.” None of these hypotheses have sufficient substantiation.
For decades TGF-β superfamily of cytokines (growth factors) has been acknowledged as inhibitors of both myoblasts proliferation and differentiation [3, 4, 23, 79]. Among several members myostatin (MSTN) also identified as growth and differentiation factor-8 (GDF-8) attracts special interest as MSTN knockout mice have unusually large shoulder and hip muscles [114, 184, 222]. “Loss of function” mutations in MSTN gene were demonstrated to enlarge skeletal muscles by increased number and size of muscle fibers in other species including human beings [71, 81, 82, 113, 164]. When secreted, MSTN protein decreases myoblast proliferation by stimulating p21 protein, a well-known cyclin-dependent kinase Cdk2 inhibitor [80, 187, 188]. Cdk2 in turn inhibits G1 to S phase transition. Molecular mechanism of MSTN activity seems to hamper satellite cell proliferation through activin type II receptor (Act RIIB) as dominant negative form of Act RIIB in transgenic mouse lines exhibited dramatic increases in muscle mass comparable to those seen in MSTN knockout mice [97]. MRF genes are transcriptionally repressed by MSTN because signal transduction from Act RIIB directly activates Smad2/3 proteins with resultant fall in MyoD and myogenin gene expression [92, 105]. Moreover, MSTN is associated with increased Smad2/3 phosphorylation levels and decline in MyoD and myogenin gene transcription [206]. Furthermore, satellite cell proliferation is noticeably elevated in MSTN-null mice [111]. MSTN is produced by skeletal muscle and adipose tissue [6], even though its expression levels are subject to regulation following muscle damage [215]. Fasting and glucocorticoids were indicated as possible MSTN gene incentives [11, 159], and both C/EBP-δ and fewer miR-27a/b seem to mediate the respective muscle wasting effects [5, 8]. Additionally, the FoxO1 and TGF-β/Smad3, MyoD, and JNK/p38 kinase pathways are involved in MSTN secretion [9, 74, 169]. The former, FoxO1-dependent muscle atrophy results from insulin resistance, as insulin inhibits FoxO1 in skeletal muscle cells [104]. Additionally, constitutive FoxO1 activity causes MSTN gene disinhibition [9] in insulin-resistant states being followed by elevated MSTN expression [7]. In contrast, MSTN is downregulated during muscle regeneration and its levels are returned to normal in mature fibers [116, 193, 197, 222]. To some extent, there is also contribution of MSTN to aberrant regeneration in mdx mice (animal model of Duchenne muscle dystrophy) as improved muscle regeneration was observed in mdx:MSTN −/− mice [202].
5.6 Myogenic Myokines
Different cytokines are produced and released by skeletal muscle, while the exact role played by each is not fully elucidated. Despite IL-6 which is fairly well described in the context of skeletal muscle as endocrine organ, IL-8 is acknowledged as potent chemoattractant for neutrophils during acute phase of inflammatory reaction [17]. IL-8 belongs to distinct family of CXC chemokines where the acronym’s C stands for cysteine while X is any amino acid that separates two cysteine residues at the NH2 terminus. Interestingly, IL-8 plasma concentration is altered by physical activity but barely moderate-to-exhaustive eccentric exercise rouses IL-8 gene expression in skeletal muscle and its blood plasma levels [125, 126]. A number of reports corroborated elevated IL-8 plasma levels following training during and after marathon run [127, 128, 133]. While accurate physiological IL-8 function in skeletal muscle is not known, some authors ponder IL-8 as locally acting and associated with mild inflammatory response associated with single muscle fiber damage. This assumption is substantiated by the miniature IL-8 observed during and following concentric muscle contractions that in general are not injurious to skeletal muscle. Some studies indicate that IL-8 is angiogenic. Summing up, IL-8 by binding to its receptor CXCR1 induces chemotactic effect, whereas it stimulates endothelial epithelium for neovascularization through CXCR2 [20, 87,130].
Besides IL-6 and IL-8, also IL-15 gene was shown to be induced in skeletal muscle during strength training [124]. The IL-15 signaling peptide acts on target cells by attaching to heterotrimeric membrane receptors (IL-15Rαβγ) with Janus kinases (JAK1 and 3) and STAT-3 and STAT-5 [65]. Since IL-15 cytokine is present as intracellular and secretory form, its detection is not easy carrying inconsistent reports [124, 125, 132, 135]. Anyway, several “in vitro” studies carried out on myogenic cells revealed IL-15 as anabolic factor capable to increase MyHC in differentiating muscle cells autonomously from IGFs [63, 151]. Similarly, this cytokine was demonstrated to have anabolic effects on skeletal muscle “in vivo” [150]. Furthermore, IL-15 participates in cross talk between muscle fibers and adipocytes [14]. It reduces adipose tissue mass with concurrent skeletal muscle overgrowth [30], and this is a unique muscle-to-fat endocrine axis with high therapeutic potential to combat muscle wasting in cachexia. The distinctive function of IL-15 is manifested in fully differentiated muscle cells where this cytokine represses proteolysis in muscle cachexia [150]. Accordingly, the IL-15 mRNA was markedly upregulated in differentiated skeletal myotubes but not undifferentiated myoblasts [152]. Summing up, IL-15 could be regarded as skeletal muscle growth-promoting myokine underestimated in prevention of muscle wasting.
5.7 Adipokines in Skeletal Muscle Growth
There is more than 30 different adipokines produced by adipocytes, making adipose tissue a significant source of regulatory factors with some being essential for fat-to-muscle communication. Leptin peptide, a “satiety hormone” is probably the most interesting amongst adipokines. It acts on target cells similarly to IL-6 as both leptin receptor (LRb) and IL-6 receptor (gp130Rβ) share a sequence homology liable for JAK recruitment and STAT phosphorylation needed for gene regulation although LRb and gp130Rβ are ligand specific without cross-reactivity [50]. Can a common signaling pathway for leptin and IL-6 evoke distinct cellular responses? The answer is yes, as IL-6 binds initially to IL-6Rα and as multifunctional cytokine exerts its biological activities through two molecules: IL-6Rα (IL-6 receptor) and gp130Rβ. When IL-6 binds to mIL-6Rα (membrane-bound form of IL-6R), homodimerization of gp130β is induced and a high-affinity functional receptor complex of IL-6, IL-6Rα, and gp130β is formed. Interestingly, sIL-6R (soluble form of IL-6R) also binds with IL-6, and the IL-6–sIL-6R complex can then form a complex with gp130β [117]. The complex activates JAKs that then phosphorylate tyrosine residues in the cytoplasmic domain of gp130β. The gp130β-mediated JAK activation by IL-6 triggers two main signaling pathways: the gp130β Tyr759-derived SHP-2 (Src homology 2 domain-containing protein tyrosine phosphatase-2)/ERK (extracellular signal-regulated kinase) MAPK (mitogen-activated protein kinase) pathway and the gp130β YXXQ-mediated JAK/STAT pathway [171]. In contrast to LRb, gp130β has more tyrosines for phosphorylation (Y767, 814, 905, 915) which is functionally important to negate feedback mechanism mediated by suppressor of cytokine signaling (SOCS) proteins [205]. SOCS3 binds to phosphorylated LRb through its SH2 domain to stop JAK-mediated phosphorylations [22] and additionally recruits ubiquitin-transferases for JAK/STAT degradation [2]. Consequently, leptin-dependent regulation of skeletal muscle is transient, whereas IL-6 seems to induce long-term effects. It was noticed that both cytokines possibly stimulate AMP-activated protein kinase (AMPK) by T172 phosphorylation mediated by AMPK kinase (LKB1) [213]. Alternatively, by increased cellular ATP turnover, leptin and IL-6 may activate AMPK with elevated levels of allosteric activator AMP. This system is fundamental for energy sensing and fatty acid oxidation in skeletal muscle, so leptin and IL-6 are mighty directors in delivery of substrates for energy production (acetyl-CoA). Recently, we observed other metabolic effects of leptin. In cultured C2C12 mouse myogenic cell line, this cytokine stimulated myoblast mitogenicity and inhibited muscle cell differentiation through JAK/STAT and MEK signaling pathway where MEK is an upstream ERK kinase (MAPKK) [145, 146]. Leptin through MEK-dependent manner caused GSK-3β phosphorylation (Y216-GSK-3β) with resultant drop in myoblast viability and fusion. Overall, more research on adipokines is required in order to shed light on fat-to-muscle influences.
5.8 Conclusions and Perspectives
Skeletal muscle fibers as permanent cells do not divide, so upon injury this organ regeneration is entirely reliant on stem cell subpopulations including satellite cells which are activated by a myriad of signals where cytokines play the prime role. Nowadays, it is widely accepted that cytokines which were initially specific growth/differentiation factors of leukocyte origin are also synthesized and secreted by other tissues including skeletal muscle (myokines). It appears, that myokine profile is dependent on the physiologic (type and intensity of exercise, muscle fiber type) or pathologic (the magnitude of injury) conditions. Thus, it is obvious the pattern of myokines controls skeletal muscle growth and decline in health and disease. Skeletal muscle growth is regulated by mitogenic and survival signals (HGF/SF, FGF, IGFs, LIF), whereas damaged skeletal muscle is characterized by necrosis and activation of pro-inflammatory (TNF-α, IFN-γ, IL-1β, IL-6) followed by anti-inflammatory cytokines (IL-1ra, soluble TNF-α receptors (sTNF-R), and IL-10) targeting muscle satellite cells and controlling skeletal muscle degeneration and regeneration phase, respectively. Finally, TGF-β superfamily of cytokines, greatly MSTN, stops further growth and muscle tissue regeneration. As skeletal muscle satellite cells are decisive for skeletal muscle regeneration, understanding the molecular mechanisms of their activation by myokines seems to be essential to combat aberrant muscle growth and repair.
Acknowledgements
Support for this work was provided by grant No UMO-2013/11/B/NZ5/03106 from the National Science Centre in Poland.
References
1.
Acharyya S, Ladner KJ, Nelsen LL, Damrauer J, Reiser PJ, Swoap S, Guttridge DC (2004) Cancer cachexia is regulated by selective targeting of skeletal muscle gene products. J Clin Invest 114:370–378PubMedPubMedCentral
2.
Alexander WS (2002) Suppressors of cytokine signalling (SOCS) in the immune system. Nat Rev Immunol 2:410–416PubMed
3.
Allen RE, Boxhorn LK (1987) Inhibition of skeletal muscle satellite cell differentiation by transforming growth factor-beta. J Cell Physiol 133:567–572PubMed
4.
Allen RE, Boxhorn LK (1989) Regulation of skeletal muscle satellite cell proliferation and differentiation by transforming growth factor-beta, insulin-like growth factor I, and fibroblast growth factor. J Cell Physiol 138:311–315PubMed
5.
Allen DL, Cleary AS, Hanson AM, Lindsay SF, Reed JM (2010) CCAAT/enhancer binding protein-delta expression is increased in fast skeletal muscle by food deprivation and regulates myostatin transcription in vitro. Am J Phys Regul Integr Comp Phys 299:R1592–R1601. doi:10.1152/ajpregu.00247.2010
6.
Allen DL, Cleary AS, Speaker KJ, Lindsay SF, Uyenishi J, Reed JM, Madden MC, Mehan RS (2008) Myostatin, activin receptor IIb, and follistatin-like-3 gene expression are altered in adipose tissue and skeletal muscle of obese mice. Am J Physiol Endocrinol Metab 294:E918–E927. doi:10.1152/ajpendo.00798.2007 PubMed
7.
Allen DL, Hittel DS, McPherron AC (2011) Expression and function of myostatin in obesity, diabetes, and exercise adaptation. Med Sci Sports Exerc 43:1828–1835. doi:10.1249/MSS.0b013e3182178bb4 PubMedPubMedCentral
8.
Allen DL, Loh AS (2011) Posttranscriptional mechanisms involving microRNA-27a and b contribute to fast-specific and glucocorticoid-mediated myostatin expression in skeletal muscle. Am J Phys Cell Phys 300:C124–C137. doi:10.1152/ajpcell.00142.2010
9.
Allen DL, Unterman TG (2007) Regulation of myostatin expression and myoblast differentiation by FoxO and SMAD transcription factors. Am J Phys Cell Phys 292:C188–C199. doi:10.1152/ajpcell.00542.2005
10.
Alvarez B, Quinn LS, Busquets S, Lopez-Soriano FJ, Argiles JM (2002) TNF-alpha modulates cytokine and cytokine receptors in C2C12 myotubes. Cancer Lett 175:181–185PubMed
11.
Anagnostis P, Athyros VG, Tziomalos K, Karagiannis A, Mikhailidis DP (2009) Clinical review: the pathogenetic role of cortisol in the metabolic syndrome: a hypothesis. J Clin Endocrinol Metab 94:2692–2701. doi:10.1210/jc.2009-0370 PubMed
12.
Anderson JE, Mitchell CM, McGeachie JK, Grounds MD (1995) The time course of basic fibroblast growth factor expression in crush-injured skeletal muscles of SJL/J and BALB/c mice. Exp Cell Res 216:325–334PubMed
13.
Anderson JE (2000) A role for nitric oxide in muscle repair: nitric oxide-mediated activation of muscle satellite cells. Mol Biol Cell 11:1859–1874PubMedPubMedCentral
14.
Argiles JM, Lopez-Soriano J, Almendro V, Busquets S, Lopez-Soriano FJ (2005) Cross-talk between skeletal muscle and adipose tissue: a link with obesity? Med Res Rev 25:49–65PubMed
15.
Armstrong RB, Warren GL, Warren JA (1991) Mechanisms of exercise-induced muscle fibre injury. Sports Med 12:184–207PubMed
16.
Asakura A, Rudnicki MA (2002) Cellular and molecular mechanisms regulating skeletal muscle development. In: Mouse development. Academic, Orlando, FL, pp 253–278
18.
Bark TH, McNurlan MA, Lang CH, Garlick PJ (1998) Increased protein synthesis after acute IGF-I or insulin infusion is localized to muscle in mice. Am J Physiol Endocrinol Metab 275:E118–E123
19.
Barton-Davis ER, Shoturma DI, Sweeney HL (1999) Contribution of satellite cells to IGF-I induced hypertrophy of skeletal muscle. Acta Physiol Scand 167:301–305PubMed
20.
Bek EL, McMillen MA, Scott P, Angus LD, Shaftan GW (2002) The effect of diabetes on endothelin, interleukin-8 and vascular endothelial growth factor-mediated angiogenesis in rats. Clin Sci 103(Suppl 48):424S–429SPubMed
21.
Ben-Levy R, Hooper S, Wilson R, Paterson HF, Marshall CJ (1998) Nuclear export of the stress-activated protein kinase p38 mediated by its substrate MAPKAP kinase-2. Curr Biol 8:1049–1057PubMed
22.
Bjorbaek C, Buchholz RM, Davis SM, Bates SH, Pierroz DD, Gu H, Neel BG, Myers MG Jr, Flier JS (2001) Divergent roles of SHP-2 in ERK activation by leptin receptors. J Biol Chem 276:4747–4755PubMed
23.
Blachowski S, Motyl T, Orzechowski A, Grzelkowska K, Interewicz B (1993) Comparison of metabolic effects of EGF, TGF-a, and TGF-b1 in primary culture of fetal bovine myoblasts and rat L6 myoblasts. Int J Biochem 25:1571–1577PubMed
24.
Bodine SC, Latres E, Baumhueter S, Lai VK, Nunez L, Clarke BA, Poueymirou WT, Panaro FJ, Na E, Dharmarajan K, Pan ZQ, Valenzuela DM, DeChiara TM, Stitt TN, Yancopoulos GD, Glass DJ (2001) Identification of ubiquitin ligases required for skeletal muscle atrophy. Science 294:1704–1708PubMed
25.
Boehm U, Klamp T, Groot M, Howard JC (1997) Cellular responses to interferon-γ. Annu Rev Immunol 15:749–795PubMed
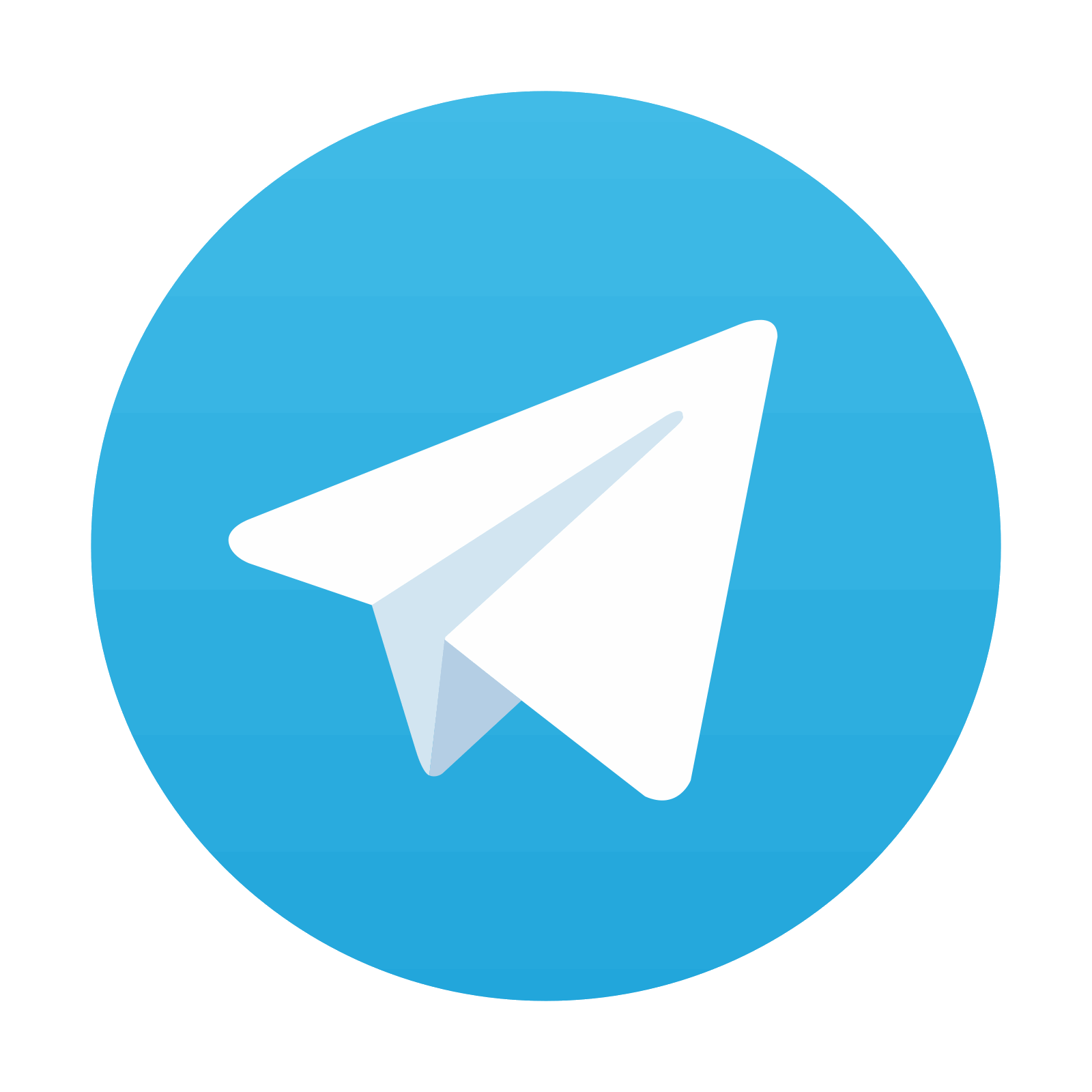
Stay updated, free articles. Join our Telegram channel
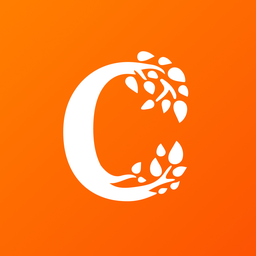
Full access? Get Clinical Tree
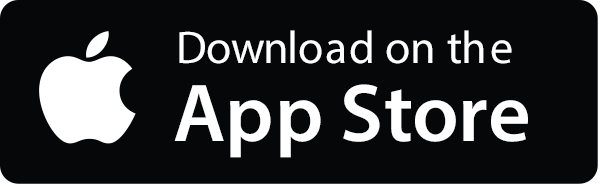
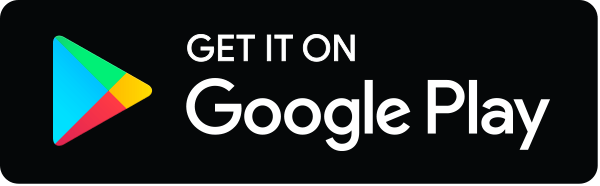