Chapter 8.3 Advanced MRI techniques for in-vivo biomechanical tissue movement analysis In the past, dynamic motion imaging utilized clinically inapplicable methods, such as 3D X-ray stereophotogrammetry that required the insertion of metal balls into the bones (Lundberg 1989). Computerized tomography (CT), with recent advances in its hardware and speed, can be utilized for the same purpose, if the focus is predominantly on the bones (Crisco et al. 1999). CT with advanced post-processing tools eliminates the need for implantable markers; however, X-ray doses become critical when multiple 3D datasets are needed. In comparison, ultrasound imaging produces relatively low resolution images for soft tissue movement analysis with modest contrast only at some of the tissue boundaries, but its use is much cheaper. MRI, without ionizing radiation effects, is ideal for repeated and prolonged experiments, routinely required in musculoskeletal research. The basic principle is that molecules (e.g., hydrogen nuclei) acting like dipoles (their top-like movement is referred to as spin) are aligned by a static magnetic field and, by dynamic high-frequency radio signals, specific molecules are given special magnetic properties which can be imaged. Since these magnetic properties are temporary, real tissue properties can be used to study dynamic change of the tissues. MRI has an additional advantage that images can be acquired at positions and orientations defined by the user, as long as the subjects are not claustrophobic and the planned movement can be performed within the scanner’s confined spaces. Ongoing efforts to provide nonclaustrophobic machines (such as open magnets) will help dynamic musculoskeletal imaging only in a limited way, since most of such systems suffer from signal-to-noise limitations due to their low static magnetic fields (yielding lower temporal and/or spatial resolution). Newer short- and wide-bore magnets with 1.5 T or higher magnetic fields provide some marginal solution, since movements are still restricted. Standing during MR imaging, most exercise routines, and weight bearing is still not common practice except with few specialized systems (Gilbert et al. 2006) or creative solutions. Most of the time, the equipment costs (both at startup and maintenance) and expertise required for its advanced utilization are the real limitations of dynamic MR imaging. Routine MR imaging is usually a slow process; image information is gathered step by step by collecting a set of signals (echoes) at different excitation scenarios. At each step, unique phase and frequency information is embedded on the individual signals, based on their physical locations, which later helps their actual coordinate identification. The combined signals are filled one echo at a time into the “k-space” and the image is obtained via Fourier transformation (Stark & Bradley 1999). Since different echoes are obtained at different times, movement of the tissues could produce significant artifacts within images. Therefore, in routine MR imaging, movement is not welcomed; for example, cardiac MRI should deal with heart and respiratory motion during the image acquisition: a first approach is acquiring consecutive images as rapidly as possible (“real-time” MRI) and the second approach is segmented k-space imaging (Haacke et al. 1999). Real-time MRI has limited spatial resolution (due to the limited number of k-space lines that can be acquired at a given time), and relatively poorer temporal resolution (typically 50–300 ms depending on spatial resolution). Improvements are achieved using echo-planar techniques (i.e., collecting several k-space lines per excitation (Epstein et al. 1999), by using longer and more exotic (e.g., spiral) k-space readout trajectories (Meyer et al. 1992) and by employing parallel imaging techniques requiring multiple receiver coils (Pruessmann et al. 1999), each with varying signal–noise-ratio (SNR) penalties. Segmented k-space imaging is used extensively in cardiac imaging, permitting the acquisition of a set of images at multiple cardiac phases over the course of several heartbeats in a single ECG-gated, breath-hold scan. The main idea is to repeat the task and synchronize image acquisition and task repetition. This is achieved by partitioning the k-space data matrix into several “segments”. The data of each k-space segment is acquired at a single repetition and successive segments are acquired in following repetitions (heartbeats). The image is effectively an average of all repetitions but could provide a series of temporal snapshots of a repetitive motion. The temporal resolution can be improved by reducing the segment size, at the price of an increased number of repetitions and total imaging time. Segmented k-space imaging techniques employ interpolation techniques to adjust for slight variations in the cyclic heart motion (Feinstein et al. 1997). Please note that heart movements can usually be considered as reliably repeatable, but this is not the case for some dynamic musculoskeletal movements. For further reading on fast imaging techniques, the reader is referred to one of several review articles, focusing mostly on cardiac implementations (Reeder & Faranesh 2000). Historically, heart movement analysis, especially of the left ventricle, has been the main model focus. Generic deformable surface models (Pentland & Horowitz 1991), models exploiting curvature information (Duncan et al. 1991), and 4D models with temporal constraints (Shi et al. 1994) have been described previously for cardiac analysis. In musculoskeletal imaging, detailed kinematical models are built using MRI-derived 3D structures. For example, the peritalar joint complex is analyzed in vivo using 3D data sets acquired during foot movement at eight positions, ranging from extreme pronation to extreme supination (Stindel et al. 2001). Similarly, shoulder kinematics is examined using sequential increments of arm endo–exorotation and comparison to 3D models of glenoid and humerus (Rhoad et al. 1998). Other examples are movement analysis of the spine (McGregor 2001), patella (Sheehan 1999), wrist (Keir 2001), or building models for general locomotion (Arnold 2000). Another set of applications of dynamic MRI involves detailed evaluation of internal movements occurring physiologically or in pathological conditions. For these applications, subjects are asked to perform certain maneuvers (e.g., straining) while inside the magnet, and newly acquired images are compared with control images (e.g., in assessment of pelvic floor defects; Rentsc 2001). Although the aim is to confirm initial diagnoses and evaluate the anatomy of defects of deeper structures, detailed quantitative analysis using the advanced techniques (previous section) could also be performed in almost all cases, when needed.
Introduction
Dynamic MRI and in-vivo movement analysis
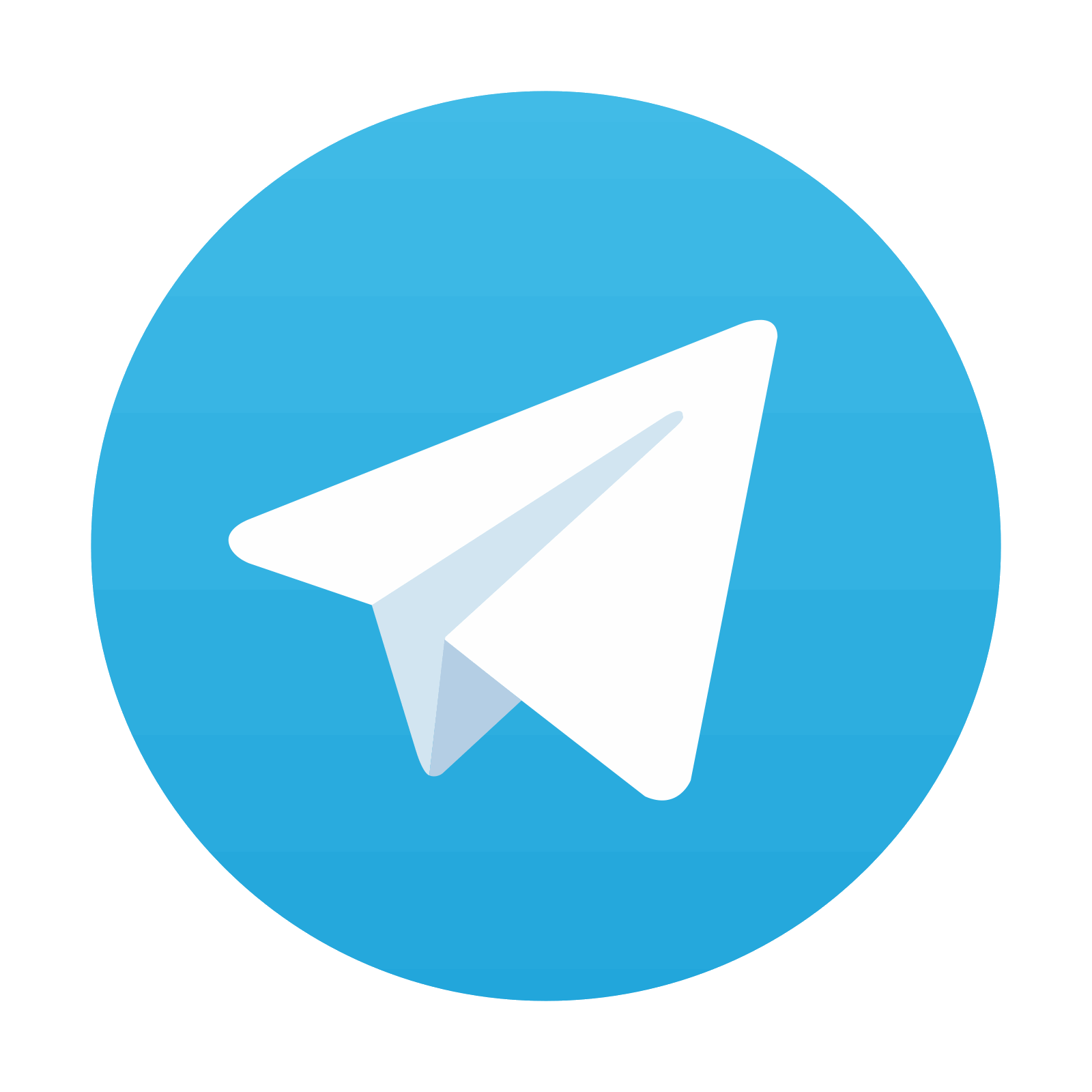
Stay updated, free articles. Join our Telegram channel
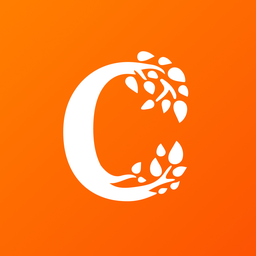
Full access? Get Clinical Tree
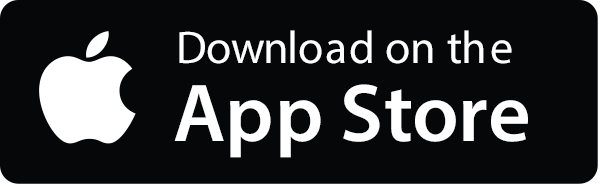
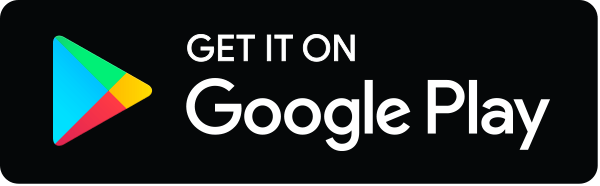