Fig. 29.1
Axial T2 color map of the middle arm in an asymptomatic volunteer. When matched to the color scale of T2 values, note that the values of the flexor and extensor muscles’ fibers are lower than those of the surrounding soft-tissues containing fat, such as the superficial and deep aponeurosis, the subcutaneous fat, and the bone marrow
It is accepted that, in different tissues the rate of interactions between water and macromolecules will vary; when interactions increase, T2 decreases, when interactions decrease T2 increases. T2 mapping has been widely applied in clinical research, mainly in the assessment of hyaline articular cartilage [26–30]. In muscles, physiologic or pathologic changes in the interactions between water and macromolecules will affect the T2 relaxation times (alterations of water binding). Furthermore, the distribution of water in the intracellular and extracellular compartments of muscles directly contributes with different components to the T2 relaxation times with this technique [17]. About 80 % of the water signal from muscles is from intracellular water (contributing to a fast relaxation time – 20–40 ms), whereas around 10 % of the water signal is from extracellular water (contributing to a slow relaxation time – 150–400 ms) [31].
It has been demonstrated that the T2 relaxation time of skeletal muscles increases during and after exercise [32–40]. The underlying mechanism for the increase is not fully understood, and probably multiple factors are involved. During exercise, contraction of muscle fibers requires energy consumption, leading to an increase in intracellular osmolites (sodium, phosphate, and lactate) responsible for an increase in intracellular osmolarity. This will cause water influx into the muscles with an increase in intracellular water, leading to an increase in T2 relaxation times [41]. Other factors are muscle perfusion [42] and a decrease in pH [43, 44]. Even though the mechanism involved in exercise-induced changes of muscles is not fully understood, the differences in T2 relaxation times between metabolically stressed muscles and less active muscles have practical applications. For specific exercises in the upper and lower limbs, as well as in the trunk, it is possible to isolate specific muscles or groups of muscles that activate during and after exercise [32, 35–38]. Sometimes, even the degree of activation (function) may be estimated as well [34]. In the literature, T2 mapping of exercised muscles is often referred as “functional MRI” or “fMRI” of muscles. Two decades ago a linear relationship between the mean muscular force produced during exercise and the T2 values of calf muscles was demonstrated; the increase in mean force correlated with the increase in T2 values [39]. The same study also tested the effect of venous occlusion on T2 values of muscles, but could not demonstrate a direct relationship between these two factors. Another study [45] showed that specific muscles around the neck demonstrated activation after specific head movements by showing an increase in T2 values, and the absolute and relative cross-sectional area of muscle exhibiting an increase in T2 values was greater when the exercise load applied was greater, providing another demonstration of a linear relationship between muscular activity/load and T2 values. T2 mapping was also shown to be correlated with quantitative measurements of muscle activity. A linear relationship between exercise intensity and T2 values was demonstrated [35], in a group of seven volunteers at rest after a series of 100 repetitions of 90-degree hip and knee flexion. Then they repeated the exercises but with a one kilogram load on the right ankle, and then they were scanned again at rest. The increase in T2 values of the right psoas muscle followed the increase in the exercise load; T2 values after the second series were greater than after the first series. A more recent study [37] assessed the correlation between total power output of muscles of the thigh during two, five, and ten sets of sprint cycling and increased T2 values of these muscles. A moderate and significant correlation was found between total power output and a linear increase in T2 values of the quadriceps. Although the thigh muscles were not uniformly activated after exercising, a linear increase in T2 values was demonstrated for the majority of them. Adams et al. [46] evaluated muscle activity in seven subjects before and after they performed five sets of concentric or eccentric arm curls with each of four resistances accounting for 40, 60, 80, and 100 % of their 10 repetition maximum for concentric curls. They did so by scanning the middle of the arm on MRI using T2 mapping, as well as by collecting surface electromyography signals from the biceps brachii and the long head of the triceps brachii muscles. Both concentric and eccentric contractions lead to an increase in both T2 and integrated electromyography, which were strongly correlated. The relationship between T2 values and muscle work rate has also been demonstrated in previous studies. Jenner et al. [43] reported the results from a study evaluating the signal intensity of the anterior tibialis muscle on MRI during progressive ankle dorsiflexion exercise, and concluded that signal intensity was linearly correlated to exercise intensity, but not with the total work performed.
One must be careful when affirming that T2 changes may determine precisely the amount of work performed by a given muscle, which would be true if a linear relationship exists between T2 values and muscle work. By using a wide range of muscle work intensities, Cheng et al. [47] demonstrated that the increases observed in T2 values during increases in exercise intensity were nonlinear, especially at low work intensities. Fleckenstein et al. [48] showed that increases in the integrated force vs. time curve and in T2 values occurred during progressive maximal voluntary handgrip contractions, and repeated analyses of variance of such increases were statistically significant. However, they found that the increase in T2 values was likely represented by a hyperbolic curve as progressively longer bouts of contractions were applied – there was a limit in the increase of T2 – and suggested the existence of a limit in the amount of water that muscles absorb from the vasculature during exertion. Furthermore, by studying the T2 values in each pixel of muscles Prior et al. [32] showed that T2 did not vary substantially among different intensities of exercise.
There are some considerations that must be taken into account regarding T2 values and muscle activity. There is evidence that increases in T2 values in muscles during or just after exercising are related to muscle activity, and such changes have the potential to show which muscles are being recruited with specific exercises (Figs. 29.2, 29.3 and 29.4). The relationship between T2 values and muscle activity/work is not always linear. Several factors are probably involved in these relationships; the specific muscle being exercised, the intensity of the exercise/load, and the physical training level of the subjects. There is no consensus about how, when or where to apply T2 mapping to determine the work performed by a specific muscle or group of muscles.





Fig. 29.2
(a) Axial T2 color map of themidsection of both legs in a young male volunteer before exercising. Note that the distribution of T2 values in the muscles (colors) are mainly symmetrical when comparing both legs. (b) Axial T2 color map in the same subject after four sessions of right (unilateral) plantar flexion. Note that the T2 values of the triceps surae muscle of the right leg are higher in comparison with the non-exercised contralateral leg. T2 values in other muscles of the right leg are also elevated but to a lesser degree

Fig. 29.3
Quantitative assessment of T2 values of muscles of the mid right leg in a young female volunteer before and after six sessions of right plantar flexion. The average of T2 values in the posterior (calf) muscles increased from pre-exercise (a; 43.88 ms ± 8.03) to post-exercise (b; 50.03 ms ± 6.32). Note also the significant change in the color-coded T2 maps of the calf muscles between A and B. There was only a slight increase in T2 values (and change in color-coded) of the extensor muscles from a to b.


Fig. 29.4
Qualitative and quantitative assessment of T2 values of muscles of both thighs in a healthy female volunteer before and after squat exercising. Qualitative assessment using T2 color-coded maps of both thighs shows a diffuse increase in T2 values of muscles after a series of squats (b) when compared to T2 values before exercise (a). Quantitative assessment of the right quadriceps muscle showed an increase of the mean T2 values after squating (d; 39.1 ms ± 14.2) when compared to T2 values before exercising (c; 35.7 ms ± 10.2) (Courtesy of Augusto P. Baffa and Marcello H. Nogueira-Barbosa, University of São Paulo at Ribeirão Preto, Brazil)
The literature, however, does show that there is a much potential for T2 mapping in sports medicine. Quantitative T2 data may provide useful information about the capacity of a muscle (or a group of muscles) to be activated by a specific exercise aimed at that muscle. This may be true in cases of chronic muscle strain/rupture in which the muscle is still functionally impaired. Even without exercising the affected muscle, quantitative T2 mapping may be useful in demonstrating early fat atrophy when routine MRI does not exhibit unequivocal findings of atrophy, since an increase in the fat content of muscles will increase their T2 (Fig. 29.5 and 29.6). Also, in post-operative joints for any reason, there are cases in which, a given muscle or a group of muscles of the limb affected will not recover its initial function during the rehabilitation and muscle strengthening process. In these cases routine imaging is often unremarkable, and the application of T2 mapping before and after exercise may bring useful quantitative information. Furthermore, the application of T2 mapping as a measure of muscle recruitment would be very useful in the follow-up of rehabilitation and muscle strengthening programs in athletes [33], as it could show roughly the efficacy of specific exercises in different regions of limbs and trunk, by demonstrating which muscles are being recruited. Finally, this could be a useful tool in the assessment of the effects of many orthoses available in many sports fields. Objective T2 quantitative data may be obtained from muscles by exercising a region where a specific orthosis is being applied for training and competition. All these possible applications of T2 mapping of muscles should be tested in future prospective and controlled studies.





Fig. 29.5
Recreational 36-year-old soccer player with an untreated complete avulsion of the left adductor longus tendon at its pubic insertion 2 months prior, demonstrated on coronal fat-suppressed T2-weighted MRI (a; arrow). The appearance of the left adductor muscle on the axial T1-weighted MRI of the left thigh is unremarkable (b, arrows). Axial color T2 mapping of both thighs without exercise demonstrates multiple foci of increased T2 values within the left adductor muscle compared with the right side (c, arrows), possibly indicating early fat atrophy of the left adductor longus muscle. Quantitative assessment of T2 values (d) of both adductor muscles shows a higher T2 value on the left side than on the right (38.45 ms ± 3.52 vs. 35.57 ms ± 3.99)

Fig. 29.6
Professional 29-year-old soccer player with a history of a strain of the long head of biceps femoris (LHBF) muscle 10 weeks prior. (a) Axial T1-weighed MRI shows mild chronic changes with thickening of the LHBF tendon at the proximal myotendinous junction (arrow), without significant changes in the adjacent muscle bulk. (b) Axial fat-suppressed T2-weighted MRI does not demonstrate signs of edema in the LHBF muscle. (c) Axial color-coded T2 map of the thigh demonstrates an increase in T2 values of the LHBF muscle (green color, arrows) when compared to other muscles in the field of view, which may be related to early fat atrophy of the muscle
29.3 Diffusion Tensor Imaging and Tractography
Diffusion tensor imaging (DTI) is an MRI technique widely applied in neuroradiology for the assessment of the orientation and integrity of white matter tracts in the brain and spinal cord [49, 50]. This technique is able to assess the anisotropy of water diffusion, and fiber tracking should be feasible using DTI, on the principle that water diffusion will be greater along the orientation of the white matter fibers than in another direction. In each imaging voxel a diffusion tensor will be reconstructed from multiple diffusion-weighted MRIs with at least six independent diffusion-encoding directions (Fig. 29.7). Once the tensor is estimated, the eigenvalues and eigenvectors provide data regarding the effective diffusivity along the orthogonal directions [51, 52]. DTI enables three-dimensional assessment and visualization of the fiber tracts; it is known as “fiber tractography”. Because of the highly anisotropic nature of muscle tissues, DTI may also be applied in skeletal muscles [17, 51–58] to assess the integrity and the orientation of muscle fibers. Two important parameters may be calculated from the generated tensor images: the fractional anisotropy, representing a measure of how directional the water diffusion is, and the mean diffusivity, which represents the average length of water diffusion along the selected directions. The fractional anisotropy is by far the most used tensor value (Fig. 29.8). Further, information on water diffusivity may be provided when assessing the apparent diffusion coefficient of tissue water, which reflects cellular membrane integrity and microcirculation/perfusion.



Fig. 29.7
To cover different possible directions of water molecules’ diffusion along the different orientations of fibers, multiple diffusion-weighted acquisitions (six in this example) using different and independent diffusion-encoding directions are applied, from which a diffusion tensor image will be reconstructed

Fig. 29.8
Assessment of fractional anisotropy in a young female volunteer without muscle injury. Assessment before (a) and after (b) six sessions of plantar flexion did not show significant changes in fractional anisotropy values (how directional the water diffusivity is) in the calf muscles (0.231 ± 0.06 before and 0.225 ± 0.05 after)
Thus, DTI and fiber tractography seem to represent a powerful tool in the assessment of the skeletal muscle microstructure in different scenarios. Previous works attempted to demonstrate its usefulness in muscle microstructure assessment. By comparing patients with injured calf muscles with subjects without calf muscle injury, Zaraiskaya et al. [51] demonstrated that fractional anisotropy values were reduced in all patients when compared to controls, while apparent diffusion coefficient values were consistently higher in patients, suggesting that DTI may be useful in assessing fiber integrity and identifying injured or disrupted muscle tissue. Sinha et al. [58] assessed the feasibility of in vivo fiber tracking of the calf muscle fibers with DTI. The orientation angles of fibers in the different muscles assessed in five volunteers were mostly equivalent to those demonstrated in previous spectroscopic studies, supporting the ability of DTI in tracking muscle fiber direction. The usefulness of DTI assessment of muscles was further demonstrated in a study that aimed to create biomechanical models of the quadriceps muscles by comparing patients with chronic lateral patellar dislocation with healthy volunteers. After tracking muscle fibers from the vastus lateralis oblique and vastus medialis oblique muscles and determining the pennation angles and the orientation of resultant force vectors, the authors found that the patients had more laterally directed predicted resultant force vectors that the control group [54]. Froeling et al. [52] could reproduce in detail the complex architecture of muscle fibers of the human forearm in five healthy volunteers using DTI and applying three-dimensional fiber tractography, including a comparison between the segmented fiber tracts obtained with DTI and animations from 20 photographs taken of each isolated muscle after cadaver dissection. The potential of DTI for demonstrating muscle injury on a microscopic level (and thus assessing microstructure of muscles) was seen in a study that assessed the correlations between DTI parameters and histologic changes (Z-band disruption) before and after 300 eccentric actions of the knee extensors on an isokinetic dynamometer [53]. The authors showed that the histologic indices of damage coincided with changes in DTI parameters (fractional anisotropy and apparent diffusion coefficient), and Z-band streaming quantified per fiber was significantly correlated with fractional anisotropy. More recently the ability of DTI to measure maximum muscle power, which is an indirect measure of fiber type distribution, was assessed in 11 soleus muscles of healthy volunteers. The study showed significant correlations between soleus muscle power and some DTI parameters such as fractional anisotropy and radial diffusivity [55].
Again here, although only few studies have explored DTI in the evaluation of muscle structure and function, we may say there is great potential for this technique in sports medicine research. One example would be where low-grade acute muscle strain is seen, without significant macroscopic fiber disruption on routine clinical imaging (ultrasound or MRI), but recovery is taking longer than expected. By assessing the microstructure of muscles (fiber integrity and orientation), DTI may be able to show that damage on a microscopic level is greater in athletes with longer recovery times than in those with shorter recovery times, even though macroscopically, in routine imaging, the severity and extent of findings do not differ between the two groups. Furthermore, it may also be useful in chronic injuries, when muscle function is not improving after rehabilitation and muscle strengthening although macroscopically, on routine imaging, the injury seems to be healed. DTI may help to identify persisting alterations in fiber orientation and integrity in these cases (Fig. 29.9). Finally, a few studies have shown that some DTI parameters are correlated with microscopic muscle disruption and with muscle power, although more studies are needed to confirm these correlations. These findings suggest the potential of this technique in tailoring rehabilitation and muscle strengthening programs for athletes, as well as in monitoring response to treatment.


Fig. 29.9
An example of fiber tracking (tractography) after applying DTI in the mid thigh
29.4 MR Spectroscopy
Unlike most imaging techniques, MR spectroscopy (MRS) provides information regarding the biochemical composition of tissues but cannot assess anatomic structure. During MRS acquisition, the signals are processed by Fourier transformation into plots of frequency of nuclear rotation versus signal intensity. The chemical shift (variation in frequency) of different metabolites will be expressed in parts per million of the field strength (represented by the x-axis) and the (peak) signal intensity of different metabolites will be represented by the y-axis. The most widely used version, 1H-MRS, can study muscle composition by demonstrating resonances from fat, water, creatine, trimethylammonium-containing compounds, and many other metabolites [17, 59]. A few recent studies showed the potential of 1H-MRS for detecting and monitoring several metabolites, including creatine and lactate, and their relationships with different phases and intensity of muscle exercise [59–62].
Because most of the muscle metabolites relevant in energy transduction contain phosphorus, 31P-MRS is better suited to assess muscular concentrations in vivo and to monitor changes over time [63]. With 31P-MRS, only relevant muscle metabolites are visible in the spectrum of signals acquired: the most signal intensities are from phosphocreatine (PCr), inorganic phosphate (Pi), and the three phosphate groups of adenosine triphosphate (ATP), which are involved in the energy metabolism (Fig. 29.10). Other visible peaks include those from phosphomonoesters and phosphodiesters. Although adenosine diphosphate (ADP) cannot be directly assessed by this technique, its concentration can be calculated indirectly using the creatine kinase reaction. Furthermore, 31P-MRS has the ability to measure the pH, which can be determined from the chemical shift difference between Pi and PCr. Thus, 31P-MRS can assess the concentrations of relevant metabolites involved in energy transduction and the pH of muscles, and it can be applied in dynamic studies to acquire kinetic data on glycolytic ATP synthesis, oxidative ATP synthesis, as well as intracellular pH regulation. It is a powerful tool for the assessment of muscle metabolism and mitochondrial activity, which can be studied in the sports medicine setting, especially through rest-exercise-recovery protocols [63].


Fig. 29.10
Spectrum of (peak) signals acquired after 31P-MRS acquisition: PCr phosphocreatine, Pi inorganic phosphate, ATP adenosine triphosphate; PME phosphomonoesters, PDE phosphodiesters (Figure modified from [63])
By knowing the physiologic changes in muscle metabolites and pH during rest, exercise, and recovery after exercise periods, it is possible then to detect metabolic abnormalities in muscles where function and work are not optimal. This approach was used in a study evaluating muscle metabolism and force production in sprint-trained runners, endurance-trained runners, and untrained subjects, using 31P-MRS. The study demonstrated important differences in force production, aerobic and anaerobic muscle metabolism in these three distinct groups, showing different patterns of PCr breakdown and recovery [64]. A recent study evaluating the same groups of subjects using 31P-MRS also showed important differences among endurance and sprint athletes and untrained subjects by assessing PCr muscle metabolism [65]. 31P-MRS could be also applied to test different training methods aimed at improving muscle metabolism and function in a sports medicine setting. An example of such application was shown in a study that, by using 31P-MRS to assess PCr recovery, demonstrated the efficacy of short-term high-intensity interval training in increasing the functional oxidative capacity in the quadriceps muscle [66]. The alterations in PCr, Pi, and pH were assessed in the quadriceps muscle in a study of healthy subjects using 31P-MRS during exercise above and below the “critical power”, representing the highest constant work rate that could be sustained by the subject without progressive loss of homeostasis (of parameters assessed). The authors could nicely identify that during exercises above the critical power, there was continuous depletion of PCr with Pi and pH changing to values observed at the termination of high-intensity exhaustive exercises (changes related to the fatigue process) [67].
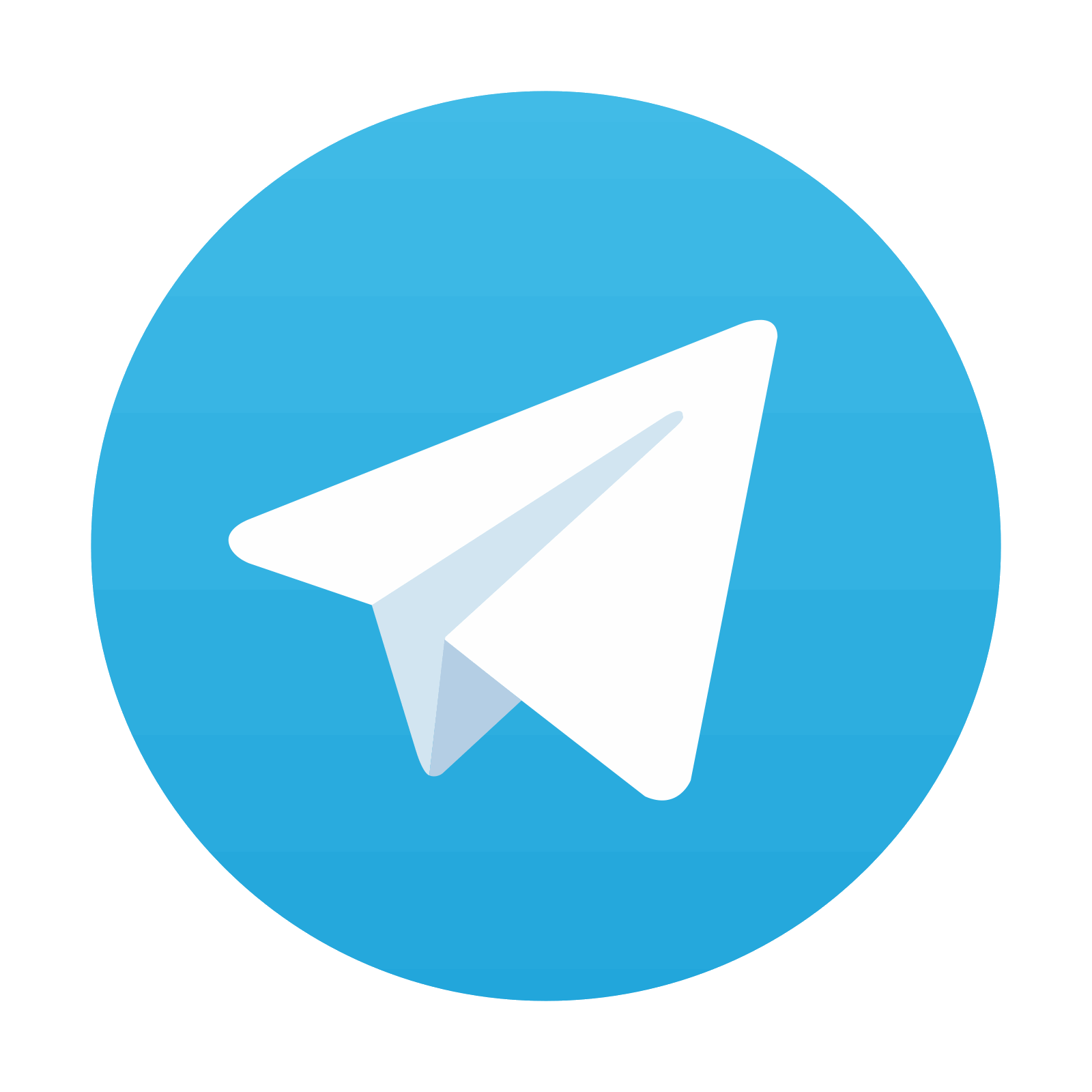
Stay updated, free articles. Join our Telegram channel
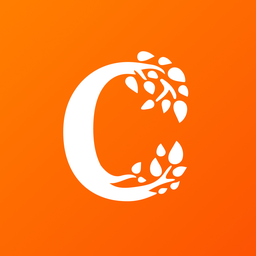
Full access? Get Clinical Tree
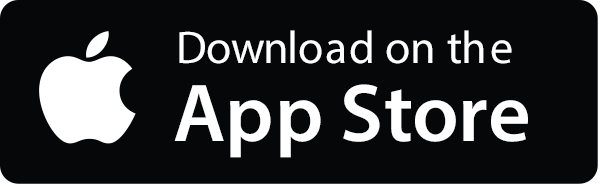
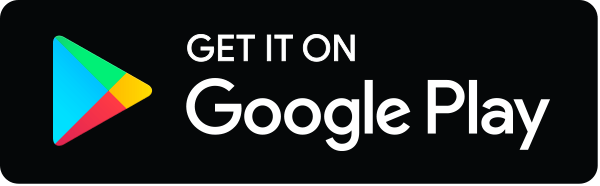