10 Mechanical Aspects of Skin
Skin has several important functions for the body; some of these are mechanical. Apart from forces acting on the teeth, external forces on the locomotor system are transmitted exclusively via the skin. Strain and deformation of the skin distribute the effect of these forces over larger areas of contact and attenuate peak forces. The ability of the skin to be readily elongated is extremely important to the functioning of joints. Any noticeable force required to elongate the skin on the convex aspect of a joint would make joint movement strenuous and decrease the range of motion. One example is the limited range of motion experienced in a case of a swollen joint. Here the skin is already strained by the swelling and tolerates only minor additional elongation. Another example is the decreased range of motion in the presence of scars close to a joint, as scar tissue is less extensible than normal skin.
Grasping and holding objects effects relatively high pressures and shear forces on the palm of the hand. A pressure distribution with peak pressure values in characteristic locations also exists under the sole of the foot. When a person is sitting or lying down, the skin is subjected to less pressure, because the gravitational force acts on a larger area of contact. In contrast to hand and foot, where we usually experience pressurization and unloading in rapid succession, the pressure on skin when sitting or lying may persist for longer periods. This may have undesirable consequences. Knowledge of the effects of pressure, tension, and shear on the skin is required for the construction and fitting of prostheses and orthoses. Deformation characteristics of the skin are of interest when planning surgical incisions and optimizing wound closure.
In addition to its mechanical functions, skin has a protective function. First and foremost, its external layer, the stratum corneum, acts as a microbial and chemical barrier. Receptors in the skin register touch, pressure, strain, and temperature. Strain effects a sensation of tension; above a certain limit, this sensation is transformed into pain. Skin plays an important role in maintaining the correct body temperature by controlling the blood flow in the skin and by regulating the production of sweat.
10.1 Anatomical Basics
Skin is composed of several distinct layers: epidermis, dermis, and subdermis ( Fig. 10.1 ). The epidermis is an avascular cellular layer. Its thickness at different locations of the body is typically 0.1 mm; on the palms of the hands or the soles of the feet a thickness of up to 1.0 mm may be encountered. Cells originate at the basement membrane, at the borderline between epidermis and dermis. Within a period of approximately 1 month these cells migrate towards the skin surface. In the course of the migration they gradually assume a flat form, lose their nuclei, and finally die.

During the migration the cells synthesize keratin, a fibrous protein. The keratin from the dead cells, reinforced by additional chemical bonding, remains at the surface of the skin and forms the stratum corneum (horny layer). At body locations which are only rarely loaded, if at all, the horny layer has a thickness in the order of 0.02 mm; at frequently and highly loaded locations the thickness can be substantially greater. During everyday life the surface of the stratum corneum is subject to continuous wear and tear. The fact that its thickness is maintained in the long run is clear proof of the continuous cellular renewal of the epidermis.
The primary function of the dermis is to provide nourishment and mechanical support to the epidermis, as its thin cellular layer is of limited mechanical strength. In terms of volume, the dermis is composed of approximately 35% collagen, 0.5% elastin, and 65% water, with small admixtures of cells and intercellular substances. The collagen fibers are identical with the tension-resistant fibers employed in tendons and ligaments. The collagen fibers form a two-dimensional mesh; in part they are arranged in preferred directions. In the unloaded state, the fibers are not straight but assume a spiral, wavy form. The form of the fibers and their arrangement in the mesh determine the elongation properties and tensile strength of the dermis. The fibers of the elastin form a second mesh. It is hypothesized that the elastin mesh is responsible for the elongation properties of skin under low stress. The intercellular substances are held responsible for the viscoelastic properties of skin.
10.2 Mechanical Properties
10.2.1 Modulus of Elasticity
Fig. 10.2 shows the stress–strain diagram of a skin sample in vitro. Starting from the unloaded state, the sample is elongated by more than 50% of its initial length under tensile stress of small magnitude (region A). After a transitional zone (region B), the strain exhibits only a small further increase in relation to the steep increase in stress (region C). When the strain approaches the value 1.0 (that is, 100% elongation with respect to the initial length), the probe eventually ruptures.

The shape of the stress–strain curve in Fig. 10.2 is qualitatively explained by the fact that in region A of Fig. 10.3 the wavy, unordered collagen fibers offer virtually no resistance to elongation.2 In the transitional region B, the fibers straighten out and become more and more aligned in the direction of the tensile force. The more the fibers are straightened and aligned, the steeper is the slope of the stress–strain curve: that is, the higher is the modulus of elasticity. With all fibers aligned, the strain can increase only by a small amount before rupture occurs. In regions B and C, the stress–strain curve of skin resembles stress–strain curves of tendons. Tendons, however, do not exhibit the large initial strain under low stress (region A), which is characteristic of skin.

Fig. 10.4 shows the initial part of the stress–strain curve of Fig. 10.2 drawn to a stress scale enlarged by a factor of approximately 100. It can be noted that, in the strain range between ε = 0 and ε = 0.4, there is a virtually linear relation between stress and strain. In this region the modulus of elasticity of skin (the slope of the curve) typically amounts to 5 kPa.2 This modulus is approximately 100 times lower than that of soft rubber. The elastic behavior of skin in the initial region of large strain is assumed to be determined not by the collagen but by the elastin mesh. In this region, where the collagen fibers are completely relaxed, the elastin is responsible for the mechanical properties of skin. The stress at the rupture limit, on the other hand, is not influenced by the elastin. 3 The elastin mesh disintegrates with age. Without elastin, deformations caused by tensile stress do not entirely return to their initial state when the stress returns to zero, and this is regarded as responsible for the formation of wrinkles.

Scar tissue contains a much higher proportion of collagen fibers than normal skin. 4, 5 Qualitatively, scar tissue is characterized by its hardness and red color. Fig. 10.5 shows the stress–strain diagram of hypertrophic scar tissue compared with normal skin. So-called hypertrophic scars develop at deep wounds, especially burns. The initial region of high strain under low stress, which is characteristic of normal skin, is absent in scar tissue. The maximum strain of scar tissue is thus less than that of normal skin. The maximum stiffness (the slope of the curves in their end region) exhibits no difference between scar tissue and skin. It is assumed that in scar tissue the collagen fibers are already aligned preferentially in the longitudinal direction of the scar. For this reason, an initial region of the stress–strain curve, where the collagen fibers align, is absent. Under high stress, the collagen fibers determine the shape of the curve, both for scar tissue and for skin.

A test which subjects a skin sample only to a single tensile force (uniaxial test) can describe the mechanical properties of skin only approximately. Since collagen forms a planar mesh, and since the strains imposed are high, a precise description of the properties of skin requires loading of the sample in two directions simultaneously (biaxial test) and documenting the strain in both directions. 6– 8 Such research helps in the solving of practical problems: for example, which procedure is best adapted to close a skin defect? Pulling the edges together? Performing additional cuts in the vicinity? Or is a transplant required?
To characterize the in vivo mechanical properties of skin, landmarks are glued on the skin. 9 The force required to increase the distance between the landmarks is measured, or perhaps the moment required to twist the landmarks against one another. Such measurements provide only qualitative data, however; specific mechanical parameters cannot be derived from them. The thickness of the tissue layer placed under strain is indeterminate; the tensile or shear stress is not uniform, and any prestress remains unaccounted for.
The so-called compression test observes the time-dependent indentation of a probe into the skin. This protocol resembles the Shore measurement of hardness employed for characterizing technical materials.
Skin is viscoelastic. If the strain of a sample of skin is kept constant, the stress will decrease in time (stress relaxation). If the stress is kept constant, the strain will increase in time (creep deformation). The viscoelastic properties of skin are assumed to be regulated by the ground substance of the dermis. The ground substance influences the internal friction between the collagen fibers and the potential shift of fluid within the dermis.
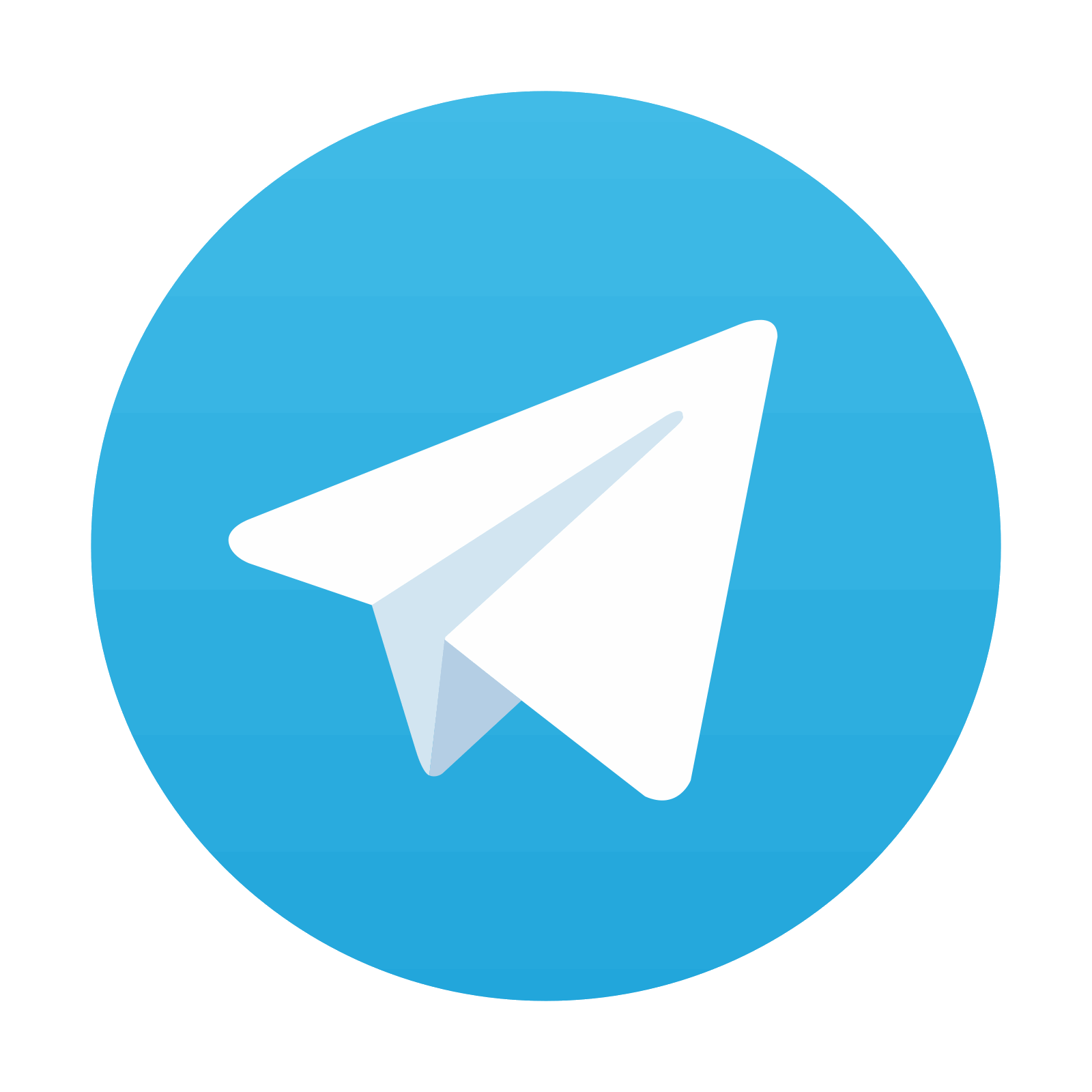
Stay updated, free articles. Join our Telegram channel
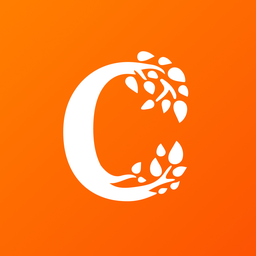
Full access? Get Clinical Tree
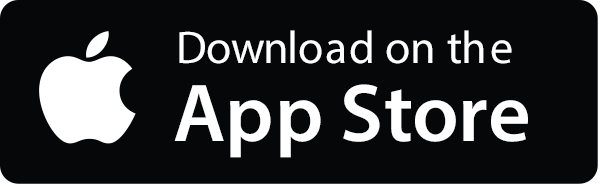
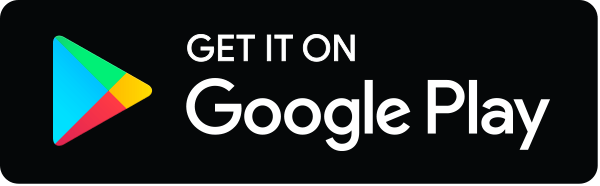
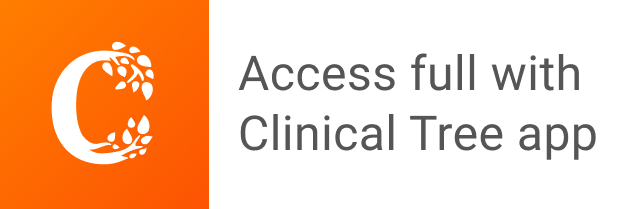