6 Review of skeletal muscle structure and function as related to cerebral palsy Basic principles of skeletal muscle contraction Basic principles of passive mechanical properties of muscle Biomechanical and neurophysiological studies of limb mechanics in spasticity Skeletal muscle in vivo morphology in cerebral palsy Skeletal muscle tissue properties in cerebral palsy Basic principles of skeletal muscle contraction The mechanical force required for voluntary motor activity is generated by skeletal muscles. This chapter provides an overview of how neurological signal is translated into the force and work that are required for motor movements. Skeletal muscle activation requires numerous proteins and molecules working in concert. When a motor neuron fires, the neurotransmitter acetylcholine is released from the motor neuron into the synaptic cleft of the neuromuscular junction. Activated acetylcholine receptors on the muscle trigger an action potential that travels across and importantly through muscle cells via the T-tubule system (Block et al., 1988). Voltage-gated calcium channels permit calcium to enter the cell when the action potential passes. Located next to the voltage-gated calcium channels within the cell are ryanodine receptors on the sarcoplasmic reticulum that respond by producing a calcium-triggered calcium release from the sarcoplasmic reticulum store. Cytoplasmic calcium binds to the regulatory machinery on skeletal muscle myofilaments inducing a translocation of the troponin/tropomyosin complex to reveal the myosin binding site on actin (Zot and Potter, 1987), collectively termed the thin filament. Force is generated when myosin from the thick filament binds the thin filament and undergoes a power stroke to displace the filaments relative to each other, as first explained by the sliding filament theory (Huxley and Hanson, 1954; Huxley and Niedergerke, 1954). A set of thick and thin filaments that interdigitate is referred to as a sarcomere and is the basic functional unit of muscle contraction. The sarcomere is bordered by Z-discs, connecting thin filaments from adjacent sarcomeres to create a myofibril from a series of sarcomeres. The myosin cross-bridge head is an ATPase, which requires ATP to release the thin filament and repeat the force generating cross-bridge cycle (Maruyama and Gergely, 1962). As calcium is pumped back into the sarcoplasmic reticulum, tropomyosin resumes its inhibitory position on the thin filament and muscle relaxation ensues. As muscle in patients with cerebral palsy has no genetic defect, these regulatory and force generating processes should remain intact. However it is possible that epigenetic modifications could impact the efficiency of translating motor neuron activity into muscle force. The motor nerve has two important methods of controlling the amount of force a muscle produces. Temporal summation is achieved when action potentials are generated sequentially in less time than is required for the muscle to relax. The faster the frequency, the greater the calcium concentration within the cell and the more force generating myosin cross-bridges that will be formed until saturating calcium is achieved at around 100 Hz (frequency) (Blinks et al., 1978). Alternatively, spatial summation is the simultaneous firing of multiple motor neurons, which, in turn, activates more muscle fibres. The more fibres activated to promote cross-bridge cycling, the greater the magnitude of force. The sliding filament theory leads to important functional implications that are inferred based on the length of a sarcomere and, thus, the amount of overlap between thick and thin filaments, referred to as the length–tension curve (Fig. 6.1) (Gordon et al., 1966). Typically divided up into three regions, the length–tension curve is based on the fact that the greater the overlap between thick and thin filaments, the greater the force a sarcomere will generate. Thus, there is an optimal length when thin filaments directly overlap the thick filaments to produce a maximal force, termed the force plateau, which is achieved at ‘optimal’ sarcomere length. As the sarcomere is stretched, less overlap exists between filaments and thus less capacity for force generation in a region denoted the descending limb. If sarcomeres are shortened from the plateau, opposing thin filaments disrupt cross-bridge formation and any shortening beyond the length of thick filament is opposed mechanically. This region is referred to as the ascending limb. The sarcomere length–tension curve is vital to understanding muscle in cerebral palsy, as clinically the muscle is often referred to as short or stretched but precisely where the muscle operates on its length–tension curve relative to normal muscle is generally unknown. Figure 6.1 The sarcomere length–tension curve for frog skeletal muscle obtained using sequential isometric contractions in single muscle fibres. The schematic arrangement of myofilaments in different regions of the length–tension curve is shown. The dotted line represents passive muscle tension. These sarcomere lengths are all based on static, or isometric, muscle lengths. To understand the force a muscle generates one must know both its length and velocity as it relates to the force–velocity curve. When a muscle undergoes a shortening, or concentric, contraction the force produced is inversely proportional to the velocity of shortening (Huxley, 1969; Katz, 1939). This is derived from the cross-bridge cycling rates, where, if filaments are sliding past each other, the cross-bridge has less chance to form. Alternatively, if muscle is activated while being lengthened (eccentric contraction), the force generated by muscle exceeds that produced isometrically. The large force generated in an eccentric contraction is largely independent of lengthening velocity, a property that is not fully understood (Harry et al., 1990). Muscle pathologies in cerebral palsy are not often viewed statically, so an understanding of how the forces are altered with motion will ultimately be critical to understanding the impairment. Spatial summation indicates that the size of a muscle is a critical component of muscle activity. Further, the arrangement of muscle fibres, termed muscle architecture, is fundamental to determining function (Fig. 6.2). The ability to produce force is proportional to the number and size of fibres in a muscle: the muscle cross-sectional area (Gans and Bock, 1965). Many muscle fibres do not run the entire muscle length from origin to insertion parallel to the action of the muscle, but are instead at an angle, termed the pennation angle. The pennation angle is used to correct the muscle cross-sectional area into physiological cross-sectional area, which is a strong predictor of force (Powell et al., 1984). Figure 6.2 Schematic illustration of muscle architectural properties in the large muscle groups of the human lower limb. Functionally, quadriceps and plantarflexors are designed for force production based on their low fibre length/muscle length ratios and large physiological cross-sectional areas. Conversely, hamstrings and dorsiflexors are designed for high excursion and velocity by nature of their high fibre length/muscle length ratios and relatively small physiological cross-sectional areas. ML, muscle length; FL, fibre length. The other important architectural parameter is fibre length, normalized to sarcomere lengths. Normalized fibre length is related to the muscle excursion, the range of lengths in which the muscle can produce force (Wickiewicz et al., 1984; Winters et al., 2011). This is especially important when investigating muscle in cerebral palsy, as contracture development severely limits range of lengths over which a muscle can produce force. Furthermore, the length of fibres and sarcomeres changes as a muscle contracts, so it is important when comparing muscles to normalize fibre lengths. This can be done by using a reference sarcomere length; however, sarcomere length is much more difficult to measure in patients than fibre lengths. Muscle architectural measurements are essentially performed in order to estimate the number of sarcomeres in parallel (physiological cross-sectional area) and number of sarcomeres in series (normalized fibre length). Various muscles have specialized architectural properties based on functional requirements for either high force capacity or high excursion ability (Lieber and Friden, 2000). For example, the quadriceps muscles have relatively short fibres and high physiological cross-sectional areas and are ideal for high forces, whereas the hamstrings have comparatively long fibres and smaller physiological cross-sectional areas that are suited for high excursion (Fig. 6.2). Our discussion of muscle so far has focused primarily on the muscle cells themselves; however, there are many other cell types present in skeletal muscle. There are, of course, fibroblasts and endothelial cells that provide the environment for muscle cells to function. Particularly in damaged or diseased muscle, there is an infiltration of many inflammatory cells that have the capacity to both impair or foster muscle regeneration (Tidball, 2005; Tidball and Villalta, 2010). The cells responsible for muscle regeneration are primarily the satellite cells, a muscle resident stem cell population named for their proximity just outside the muscle fibre but beneath the basal lamina (Fig. 6.3). Satellite cells have been shown to migrate to sites of muscle damage and divide into both a cell to replenish the satellite cell pool and a myoblast that differentiates and incorporates into an existing muscle fibre or fuses with other myoblasts to form a new myotube and eventually muscle fibre (Mauro, 1961; Yablonka-Reuveni, 2011). It is the overall interactions among these cell populations that create the pathologic state of muscle seen in muscle contractures of patients with cerebral palsy. Figure 6.3 Schematic diagram of skeletal muscle development. (A) Primitive cells differentiate into myoblasts. (B) Myoblasts fuse together to form primary myotubes. (C–F) Later, secondary myotubes arise beneath the basal lamina of the primary myotubes. Fusion of myoblasts radially and longitudinally results in the formation of the muscle fibre beneath the basal lamina (shown in cross-section in E through H). In addition, some unfused myoblasts remain as satellite cells, which are maintained in the mature tissue. (G) As the muscle matures, primary and secondary myotubes separate, each with myonuclei and satellite cells, to become a mature fibre. (H) Finally, as the muscle fibres grow, they become arranged as tightly packed polygonal cells, characteristic of adult muscle. The material above describes how skeletal muscles produce force and perform work when activated by the nervous system. However, muscles are also capable of bearing considerable passive tension, without cross-bridge cycling, in response to stretch (Fig. 6.4). Thus, a passive length–tension curve is superimposed upon the active length–tension curve described above. Muscle passive tension limits range of motion in some joints, such as the hamstrings, which limit knee extension with the hip flexed. This is especially prevalent in patients with cerebral palsy as muscle passive tension results in debilitating joint contractures. The origin of this passive tension has been controversial and is not equal in all muscles. A seminal study in the field showed that, in frog muscle, most passive tension was borne within a single myofibril (Magid and Law, 1985). This suggested that, in frog muscle, collagen, intermediate filaments and cytoskeletal structures did not play a large role in bearing passive tension. It also mitigated the impact of other extracellular matrix components in contributing to passive tension of whole muscle. Figure 6.4 Structural hierarchy of skeletal muscle. Whole skeletal muscles (here a biceps muscle is shown) are composed of numerous fascicles of muscle fibres. Muscle fibres are composed of myofibrils arranged in parallel. Myofibrils are composed of sarcomeres arranged in series. Sarcomeres are composed of interdigitating actin and myosin filaments. Hexagonal array of interdigitating myosin and actin filaments which comprise the sarcomere. The myosin filament is composed of myosin molecules, and the actin filament is composed of actin monomers. Arranged at intervals along the actin filament are the regulatory proteins troponin and tropomyosin. Cross-sections through different portions of the sarcomere reveal the interdigitation of the filament systems. This type of sectioning experiment was used to define the mechanism of muscle contraction. (A-A band, I-I band, Z-Z band). Until the giant protein titin, the largest known protein, was discovered and shown to span half of the sarcomere from the Z-disc to the middle of the thick filament and provide a physical link between filaments (Horowits et al., 1986), it was unknown what could bear passive load within the sarcomere in the absence of cross-bridges. This made it a prime candidate for being responsible for the passive tension generated within the myofibril. Indeed experiments where titin was enzymatically removed resulted in fibres that were very compliant (Funatsu et al., 1990). Furthermore, it was demonstrated that titin had many splice variants with much of the variation in the length of the extensible PEVK (rich in proline, glutamate, valine and lysine) region. Studies showed that the size and corresponding length of a titin molecule was roughly related to the stiffness, with shorter isoforms being stiffer (Freiburg et al., 2000; Horowits, 1992). This presented strong evidence for titin bearing considerable muscle passive tension. It was thus possible the titin isoform was shifted to a smaller molecule in muscle of patients with cerebral palsy, contributing to the increased passive tension. Later experiments showed that titin located in mammalian tissue such as rabbit did not bear as much of the passive load compared to frog muscle (Prado et al., 2005). In this case the primary components responsible for load bearing were found to be extracellular. The extracellular matrix is a scaffold linking together muscle fibres on multiple levels. The endomysium surrounds individual fibres, the perimysium surrounds groups of fibres called fascicles, and the epimysium is a layer of connective tissue surrounding whole muscle. The extracellular matrix consists of many components, including collagens and proteoglycans. Collagen is the primary component of extracellular matrix in muscle thought to be responsible for much of the extracellular matrix stiffness (Borg and Caulfield, 1980; Duance et al., 1977; Gillies and Lieber, 2011). Changes to the extracellular matrix may be prevalent in cerebral palsy as the muscle is clinically often referred to as fibrotic (see below). The passive tension in skeletal muscle is not purely a function of stretch (Fig. 6.5). Indeed, a stretched muscle will immediately resist stretch with a high force, followed by a period of relaxing stress to a lower steady state. This stress–relaxation principle makes muscle a viscoelastic material where the speed and time course of stretch are important components in determining passive tension (Magnusson, 1998; Meyer et al. 2011; Wang et al., 1993). These additional parameters have complicated models of passive tension in skeletal muscle (Best et al., 1994; Morgan et al., 1982). It is important to remember that passive muscle tension is not static in vivo just as one must consider the force–velocity curve when examining active tension. For example, during gait, as the quadriceps extends the knee, the force required to lengthen the hamstring is much greater than if the knee is very slowly extended. This property of passive tension is seen in cerebral palsy as the knee extension during a typical crouch gait is less than knee extension during passive stretching of the hamstring. Figure 6.5 Passive stress of myofibril elements and ECM elements, and the average passive stress in the simulated fibre superimposed on the sarcomere length–tension diagram. Myofibril passive stress, ECM passive stress and the sarcomere length–tension relationship were estimated from the work by others. Skeletal muscle is a very adaptive tissue and subject to remodelling of the properties discussed previously, based on the pattern of use. Muscles that are subjected to high stress respond by undergoing muscle hypertrophy and increasing physiological cross-sectional area. Studies have shown that eccentric contractions, which produce the highest stresses, elicit the largest hypertrophy signal (Hather et al. 1991; Roig et al., 2009). It is possible for muscles to increase or decrease their physiological cross-sectional area by either changing the number of fibres or the size of present fibres. However, after development, muscle hypertrophy in mammalian muscle is accomplished primarily by fibre hypertrophy rather than the addition of new muscle fibres (Taylor and Wilkinson, 1986). Eccentric contractions of high stress are also capable of inducing muscle damage and injury. Repair of injured muscle often results in a fibrotic response (Kaariainen et al. 2000; Serrano and Munoz-Canoves, 2010). The tipping point between muscle contractions to maintain or increase mass and those that induce injury is critical. This is exaggerated in cerebral palsy where muscles have an altered capacity for growth and regeneration. Conversely, muscles that are not subject to use undergo atrophy, as in the cases of limb immobilization, bed rest, or space flight (Lecker et al., 2004). Muscle atrophy is not just the opposite of hypertrophy, but an active process in which proteins are targeted for degradation in order to conserve the high-energy demand required for muscle upkeep. In order for muscle to effectively atrophy, proteins are ‘marked’ for degradation by ubiquitin, which is attached by a class of proteins termed ubiquitin ligases. Muscle that is actively undergoing atrophy has two ubiquitin ligases that are expressed to specifically mark contractile proteins for degradation (Bodine et al., 2001). The activation of muscle atrophy is often accompanied by muscle fibrosis as well, which occupies part of the space vacated by muscle fibres. Improper activation of the atrophy programme could compromise muscle in cerebral palsy. Muscle plasticity is often referenced in relation to a change in fibre types. Muscle fibre types have important functional consequences for the muscle. Human muscle has primarily three fibre types, which are based largely on the myosin heavy chain isoform expressed. Type I, or slow, fibres have slower cross-bridge cycling rates and produce less force than other fibre types. However, type I fibres are highly oxidative and capable of repeated contractions with very little fatigue. Type IIa fibres have faster cross-bridge cycling rates and are capable of producing larger forces than type I fibres. They still maintain oxidative metabolism and are relatively fatigue resistant. Type IIx are the fastest and most powerful fibres in human muscle. However, they have very low oxidative capacity and rely upon glycolytic metabolism for energy demands and are thus very susceptible to fatigue. Each fibre from a motor unit consists primarily of the same fibre type. This allows a sequential activation where slow motor units dominate repetitive low force contractions and then fast fibres can be recruited for periodic high force contractions (Buller et al., 1960a, 1960b). The proportion of fast and slow fibres varies across muscles, depending on their function (Fig. 6.6). With altered use it is possible to shift the proportion of fibre types within a muscle, with overactivity leading to a slower phenotype and decreased use leading to a faster phenotype. The potential magnitude of these changes in humans is controversial. However, as we have seen, the activation patterns in cerebral palsy are altered and indeed may represent a signal that is capable of inducing substantial fibre type plasticity in muscle of cerebral palsy patients. Figure 6.6 Light micrographs of the (A) vastus lateralis, (B) vastus medialis and (C) rectus femoris muscles. The top panel represents micrographs from normal muscle, and the bottom panel represents micrographs from immobilized muscles. All micrographs were taken at the same magnification. Fast fibres appear dark, and slow fibres appear light. Calibration bars=100 μm. [Reprinted with permission from Lieber, R.L., Friden, J.O., Hargens, A.R., Danzin, L.A., Gershuni, D.H., 1998. Differential response of the dog quadriceps muscle to external skeletal fixation of the knee. Muscle Nerve 11, 193–201, with permission from John Wiley & Sons, Inc.] Muscle is not only capable of adding sarcomeres in parallel with increased force but also of adding sarcomeres in series to create longer fibres and thus achieve greater excursion. Much of the research in this area was performed on cats, showing that when muscles are immobilized in a shortened position they reduce the number of sarcomeres in series and when they are placed at longer lengths they add sarcomeres in order to stay at the same point on the sarcomere length–tension curve (Tabary et al., 1972). This property of muscle is important during skeletal development as limbs extend the length of muscles, and also during surgical operations in which muscle length is altered (Boakes et al., 2007). We have seen, however, that changing muscle length with immobilization or surgery often is associated with other muscle plasticity adaptations that complicate models. Particularly, pathologic muscles may not demonstrate the ability to natively adapt the number of sarcomeres in order to maintain optimal sarcomere length. Muscle in cerebral palsy is often referred to as ‘shortened’, bringing up the question as to whether this muscle is capable of plastically changing length and serial sarcomere number. Skeletal muscle in patients with cerebral palsy is often referred to as ‘spastic muscle’. Spasticity is classically defined as a velocity-dependent resistance to stretch, with which there is an active response of muscle to resist stretch, or a catch (Lance, 1980). A spastic muscle may not have any actual muscle pathology, but instead be the result of hyperreflexia in the nervous system. It can be difficult to separate spasticity from intrinsic changes to the muscle itself. Previous studies have been conducted to delineate the passive, intrinsic and reflex-mediated increases in stiffness of spastic muscle in multiple sclerosis patients. While these patients did not have cerebral palsy, their results likely extend to cerebral palsy as well. The acquisition of these data can be complex, with the use of dynamometry, electromyography and nerve stimulation combined with signal processing methods to tease apart the intrinsic stiffness of the muscle from the overall muscle stiffness measured, which includes a reflex component (Fig. 6.7) (Lieber et al., 2004). An increase in reflex-mediated stiffness can be observed but interestingly, both studies also implicate the passive muscle stiffness as well (Mirbagheri et al., 2001; Sinkjaer and Magnussen, 1994). This increase in passive stiffness dominates particularly at the end of the range of motion where passive tension is highest. This indicates that the passive biomechanical properties of the muscle are being altered in cerebral palsy to increase joint stiffness, particularly in the case of fixed contracture where there is no reflex contribution, but joint motion is limited by increase in muscle stiffness. This is readily apparent in muscle lengthening surgeries of cerebral palsy patients, where the patient under general anaesthesia without reflex activity has a joint contracture that is immediately relieved upon muscle or tendon lengthening. Figure 6.7 Example of a mechanical torque curve measured in a human subject during mechanical perturbation of the ankle and during electrical stimulation. In performing this experiment, plantarflexor muscles are first voluntarily activated to a preset level, in this case, about 5 Nm (‘background torque’), and then the ankle is rapidly dorsiflexed at a known time (down arrow). This results in the ‘total torque’ trace, which is due to both intrinsic muscle properties as well as the reflex activation of the muscle due to the stretch reflex elicited (thick line). In a separate experiment, using neuromuscular electrical stimulation of the triceps via the tibial nerve, plantarflexion torque is again measured. But, this time, the torque recorded is only due to the intrinsic torque generated by muscle contraction, with (presumably) no neural component (thin line). Stiffness is calculated from these data in terms of torque/angle in units of Nm/degree. The zero torque level (0 Nm) is shown by the dotted line. Calibration bars are shown to the left of the figure. [Adapted with permission from Sinkjaer, T., Magnussen, I., 1994. Passive, intrinsic and reflex-mediated stiffness in the ankle extensors of hemiparetic patients. Brain 117, 355–363, with permission from Oxford University Press.]
Skeletal muscle changes due to cerebral palsy
Review of skeletal muscle structure and function as related to cerebral palsy
Basic principles of passive mechanical properties of muscle
Basic principles of plasticity in muscle
Biomechanical and neurophysiological studies of limb mechanics in spasticity
Stay updated, free articles. Join our Telegram channel
Full access? Get Clinical Tree
Skeletal muscle changes due to cerebral palsy
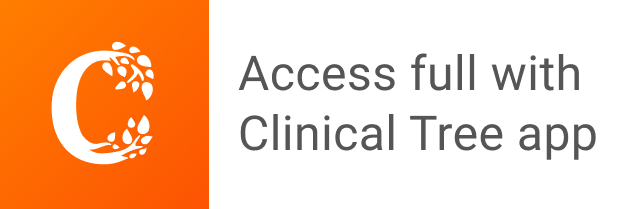