Fig. 32.1
Bioprosthetic glutaraldehyde-treated vascular grafts
Soft Tissue Augmentation Devices
Recent developments in orthopedic soft tissue reconstruction have involved the development of xenograft tissue augmentation devices. These materials are subjected to a rigorous washing process that strips the tissue of many cellular components; however, the process does not remove α-Gal epitopes. They are designed to augment existing structures and are subsequently replaced by host tissue in a relatively short time frame. Upon implantation, the device elicits a robust foreign body reaction, both to the α-Gal epitope and other proteins, resulting in an inflammatory process and replacement with fibrotic (scar) tissue that does not replicated the normal tissue [1, 23]. The clinical utility of the device is limited by the short residence time of the material mediated by inflammation and rapid post-implantation decline in device mechanical integrity. Figure 32.2 illustrates soft tissue augmentation device treatment and host response.


Fig. 32.2
Soft tissue augmentation devices
More current treatments with α-galactosidase enzyme are used permanently to remove α-Gal from porcine connective tissues. Studies in primates showed a >95 % reduction in immunological response through removal of the α-Gal epitope [13, 31]. Enzymatic elimination of the α-Gal epitope is not effective in the living organs frequently studied for transplantation due to the continual synthesis of the epitope by transplanted cells. However, since treated devices do not contain any live cells, the enzymatic depletion of α-Gal epitopes is permanent.
There are many secondary epitopes that elicit a low-level immune response. The immune-mediated destruction of the devices depleted of α-Gal epitopes is further minimized by treatment including glutaraldehyde cross-linking followed by end-capping, freezing, and irradiation. Because primary host immunological response has been attenuated by the use of the enzymatic treatment, we were able to use significantly lower glutaraldehyde treatment levels than those used in other devices.
The devices that result from this combination of treatments are very different from traditional static bioprosthetics. The reduced processing provides a device that is more biocompatible and biomechanically superior. Most importantly, the low-level treatments do not block migration of host cells into the device, gradually replacing the implanted device with a new tissue. Thus, as described below, macrophages can penetrate the device, albeit at a slow enough pace that enables the remodeling by host cells (e.g., blood vessels and fibroblasts) that infiltrate via the pathways formed by these macrophages. Reestablishment of original tissue morphology is a tremendous advantage since the patient’s own body repairs the graft from cumulative wear. Immunochemically modified tissue-derived devices provide biomechanical support and functionality to the patient immediately upon implantation and throughout the period of regrowth of the patients’ own tissue (ligamentization) [11, 21].
32.6.2 Xenograft Processing Overview
Based on the considerations above, a focused effort was undertaken to develop a xenograft ligament device from a section of the porcine patellar tendon with bone blocks and based on the immunogenicity mechanisms learned from the previous series of primate studies. Grafts were sourced from a porcine stifle and processed into a bone-tendon-bone (BTB) configuration. The graft was exposed to a series of chemical treatments. First, decellularization treatment to remove intact porcine cells and cellular components, followed by exposure to recombinant α-galactosidase enzyme solution to cleave α-gal epitopes from the graft. Removal of these α-gal epitopes was confirmed by ELISA testing with a monoclonal anti-Gal antibody that demonstrated that essentially 100 % of these epitopes were removed from the tendon portion of the construct. Following enzyme treatment, low-level glutaraldehyde cross-linking treatment was employed, with the intention of attenuating the anti-non-Gal-mediated destruction by the host immune system. We determined empirically incubating tendons in low-level glutaraldehyde followed by glycine quench of aldehyde yields for optimal conditions for macrophage infiltration allowing for the gradual remodeling of implants.
The final stage of treatment included packaging and exposure of the hydrated graft to 17.8 kGy of e-beam irradiation, a low level of irradiation intended to provide graft sterility while minimizing the degradative effects of radiation.
32.6.3 Biomechanical Evaluation
In order to mechanically characterize treated and sterilized porcine grafts, we used clinically relevant controls for comparative biomechanical evaluations and used standardized static testing methods. The two test groups included treated porcine device (pPT) and human bone-patellar tendon-bone allograft (hPT) cut to 9-mm width. Ten grafts were used in each assessment group. All testing used fresh-frozen grafts stored frozen and thawed just before testing. Porcine ligament graft specimens were immunochemically processed, while human patellar tendon grafts were sourced from accredited tissue banks as humans use graded specimens.
Structural properties were determined from load displacement curves: ultimate load, yield load, ultimate displacement, yield displacement, and axial stiffness. Axial stiffness was calculated from linear slope. Conversion of these tensile properties was accomplished by normalization of stress vs. strain plots and specimen cross-sectional area. Structural and material properties were derived for all specimens. A retrospective comparison of our porcine and human patellar tendon results from Noyes 1976 study evaluating young and old human anterior cruciate ligaments is shown for physical, structural, and material properties [27]. The biomechanical characterization of the specimens compared processed xenograft with human allograft. Human ACL construct groups are presented from Noyes 1976 study. Cross-sectional area and bone-to-bone length of the tested graft groups closely approximate the ACL. The structural properties of ultimate load, yield load, ultimate displacement, yield displacement, and stiffness in ten grafts are presented in Table 32.1.
Table 32.1
Structural properties of biomechanical tensile test groups
Porcine patellar tendon treated (pPT) (n = 10) | Human patellar tendon (hPT) (n = 10) | Human ACL (16–26 years) (n = 6) [26] | Human ACL (48–86 years) (n = 20) [26] | |
---|---|---|---|---|
Ultimate load (N) | 1,889 ± 252 | 1,387 ± 299 | 1,730 ± 660 | 734 ± 266 |
Yield load (N) | 1,437 ± 256 | 1,101 ± 397 | 1,170 ± 750 | 622 ± 283 |
Ultimate displacement (mm) | 20.5 ± 5.5 | 15.1 ± 4.5 | 11.8 | 8.3 |
Yield displacement (mm) | 14.0 ± 4.3 | 11.7 ± 3.6 | 6.9 | 6.0 |
Stiffness (N/mm) | 184.2 ± 34.8 | 181.9 ± 79.5 | 182 ± 56 | 129 ± 39 |
No significant differences were found between xenograft device and human patellar tendon test groups in the structural parameters of yield load, ultimate displacement, yield displacement, stiffness, or yield strength. No significant differences were found between these test groups in the material parameters of ultimate strain, yield strain, or modulus. The xenograft device exhibited significantly greater ultimate load, ultimate strength, and yield strength as compared to human patellar tendon grafts. Xenograft device material property results compare favorably to ACLs of young donors as reported in the literature and resulted in an activity force limit 1.3 times the ultimate strength of young donor ACLs [26].
32.6.4 Primate Anterior Cruciate Ligament Reconstruction
An ACL reconstruction study in primates was performed in 20 rhesus monkeys to evaluate the feasibility of the graft as an ACL reconstruction graft [34]. Testing involved a unilateral primate ACL reconstruction model with 2-, 6-, and 12-month sacrifice time points and clinical, histological, and biomechanical assessments. Control groups included primates implanted with an unprocessed porcine graft and allograft-implanted primates. Evaluation methods included objective functional assessment in life, radiology, serological testing of anti-Gal and anti-non-Gal antibody response, and clinical and serum chemistry. Post-sacrifice assessments included gross pathology, organ histopathology, implant histology, and postmortem biomechanics. All animals returned to normal function by 7 weeks postoperatively. Range of motion and laxity were assessed at 6 and 12 months by manual manipulation and comparison to contralateral, unoperated limbs. All range of motion and laxity measurements were considered to be clinically acceptable considering the surgical and anatomical complexities of the small primate knee. No clinically significant differences between allograft and treated porcine grafts were noted in either clinical end point. Two-month histology presented in Figs. 32.3, 32.4, 32.5, and 32.6 demonstrated parallel development between rhesus autograft and treated porcine groups in contrast to frank rejection in the untreated porcine group.





Fig. 32.3
Treated porcine ACL reconstruction device

Fig. 32.4
(a–c) Rhesus allograft histology, 2 months. (a) Intra-articular portion of the graft showing peripheral synovial formation and collagen fiber alignment (H&E, 10×). (b) Fibroblastic reorganization of graft with modest fibrohistiocytic infiltration (H&E, 40×). (c) Femoral bone tunnel showing graft integration and host bone remodeling (toluidine blue, 10×)

Fig. 32.5
(a–c) Untreated porcine patellar tendon histology, 2 months. (a) Granular organization of the native intra-articular graft periphery without residual identifiable porcine elements (H&E, 20×). (b) Cellular infiltrate of lymphocytic cells (H&E, 20×). (c) Femoral bone tunnel showing considerable graft resorption by numerous giant cells and plasma cells with original tunnel margins indicated by the dotted red line (toluidine blue, 10×)

Fig. 32.6
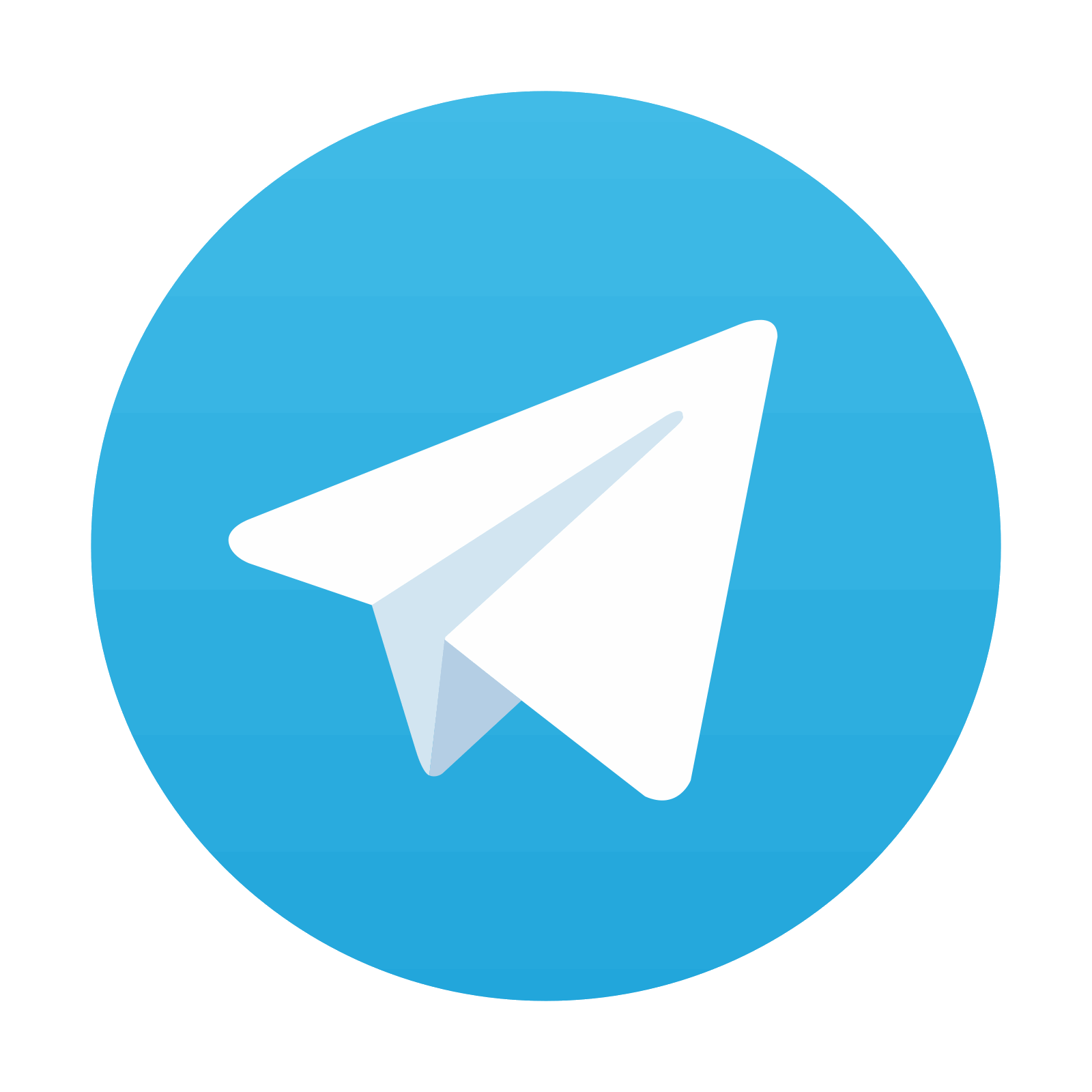
(a–c) Xenograft device histology, 2 months. (a) Peripheral synovial formation and fibrovascular organization of the graft (H&E, 20×). (b) Fibroblastic remodeling in alignment with the collagen fibrils (H&E, 40×). (c) Femoral bone tunnel showing graft-to-host bone integration and new bone formation around the edge of the tunnel (toluidine blue, 10×)
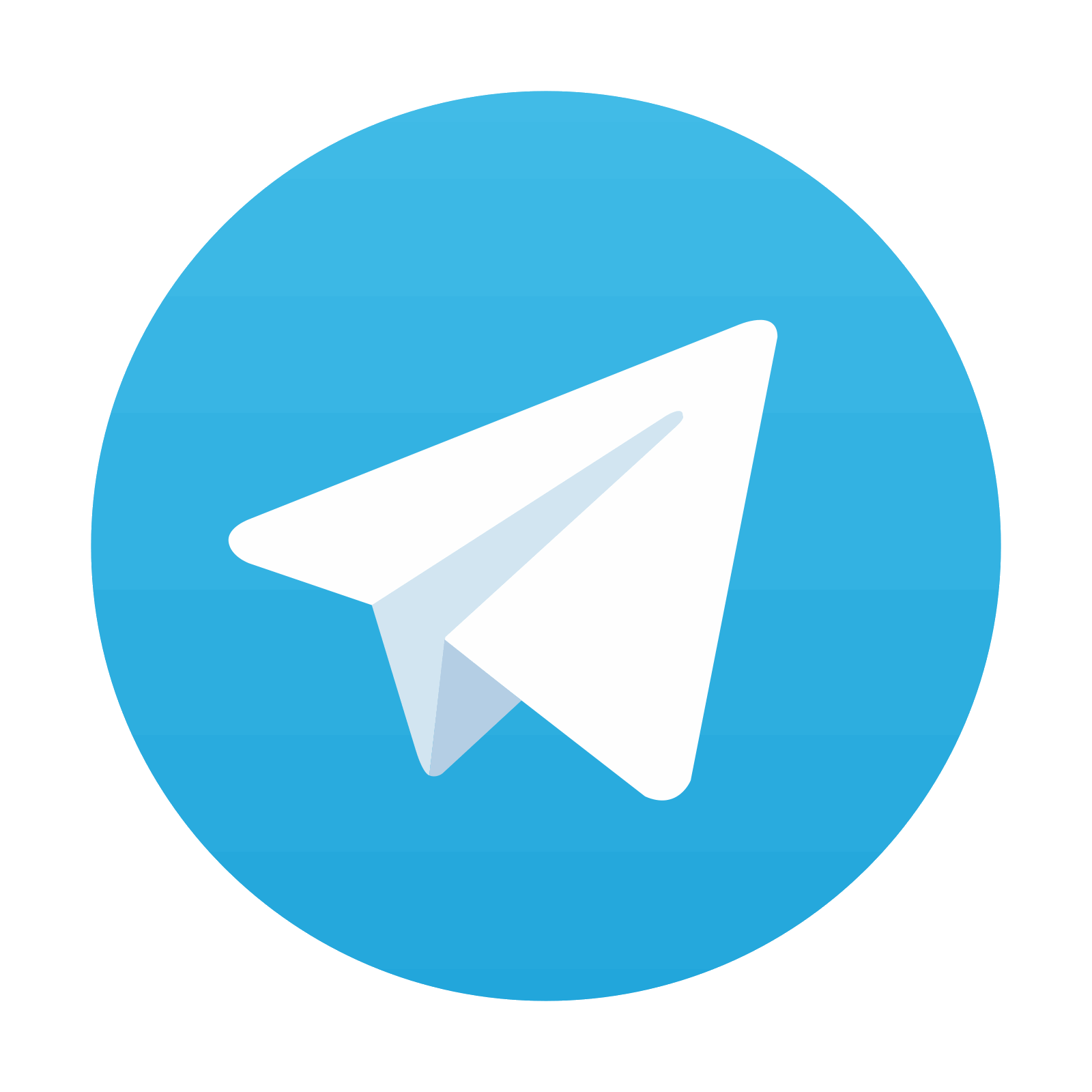
Stay updated, free articles. Join our Telegram channel
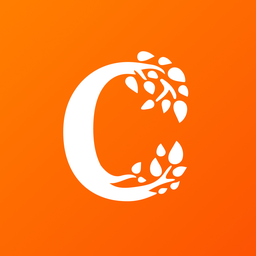
Full access? Get Clinical Tree
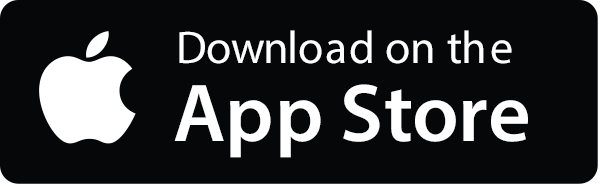
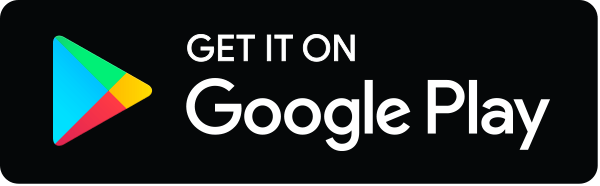
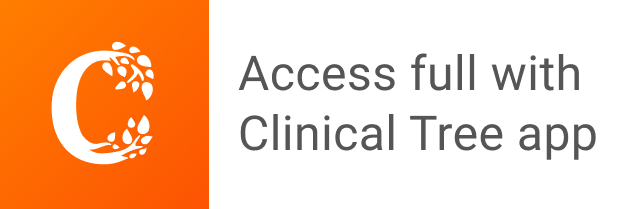