Fig. 8.1
Left: illustrates displacement of a lateral tibial plateau fracture model used to assess the effect of displacement on observed contact stresses. Right: shows that greater joint displacement leads to higher contact stresses affecting progressively smaller areas of articular cartilage. Reproduced from Brown, TD et al: Contact Stress Aberrations Following Imprecise Reduction of Simple Tibial Plateau Fractures. JOR. 1988; 6:851
Effect of Fracture on Chondrocyte Viability
Tochigi et al. impacted intact human cadaver ankles with a transarticular load of 50 J to generate tibial plafond fractures via a drop track [10]. Following impact, osteochondral fragments were sampled and cultured up to 2 days. Chondrocyte death was again reported to be higher along the fracture-edge compared to non-fracture regions in the joint. Unfortunately, there were no controls in these experiments to understand the effects of specimen handling on chondrocyte death. To date there are no studies that have specifically quantified initial injury severity and then assessed the extent of arthritic changes in an articular fracture model.
Limitations of in vitro models with cadaver joints are that it is not possible to fully elucidate the mechanobiologic response of joint tissues to injury and potential healing over time. In vivo animal models allow for such investigations. Various species of animals have been used for cartilage impact models, effect of articular cartilage incongruity via osteotomy models, or impact with articular fracture models.
Animal Models of Joint Impact Without Fracture
Articular fractures mostly likely involve not only disruption of the articular surface but also impact to cartilage at supraphysiologic loads. Defining precise loading parameters which induce arthritic changes is a challenging problem. In vivo cartilage contact biomechanics have reported cartilage strains in the knee to be 20 % in normal knees and 29 % for ACL-deficient knees [11]. Additional quantification of physiologic loading of the knee joint, for example, has been best reported with instrumented total knee replacements. Peak axial loads ranged between 2.2 and 2.8 times body weight (BW) during walking and up to 3.0 times BW with stair climbing [12–15]. Radin et al. looked at factors that affected the peak coefficient of friction seen by native joint during impact loading. They determined that combined oscillations under load with additional intermittent impact loading of bovine phalangeal joints resulted in sequential wear of cartilage and bone with nearly doubling of the coefficient of friction after 196 hours [16]. The authors concluded that the bone spared the articular cartilage during supraphysiologic impact loading.
The connection between magnitude of load and/or strain to the articular cartilage and arthritis development has yet to be established clinically. However, there are a range of animal models which have examined the effect of impact loading to articular cartilage. The majority of studies investigating impact loading of cartilage, as it relates to joint trauma and post-traumatic arthritis development, can be divided into three categories: (1) animal models of repetitive impact loading on joint tissues; (2) animal models of a single-impact load to a weight-bearing joint; and (3) animal models of a single-impact load to a femoral condyle. The results of these investigations are summarized below.
Impact Without Fracture: Animal Models of Repetitive Impact Loading
Several early studies investigated the role of cyclic loading on the joint tissue, as would be experienced with repetitive loads, for example as experienced by drill operators. In 1972, Simon and Radin reported that repetitive impact loading to the guinea pig knee resulted in joint degeneration within 3 weeks, with degenerative changes in both the subchondral bone and articular cartilage [17]. Radin et al. then reported similar findings with cyclical loading of the rabbit hind leg for 1 h daily [16]. Stiffening of the subchondral bone along with trabecular microfractures was first reported between days 4 and 11, followed by synovial changes at day 16, and then cartilage degenerative changes starting at the surface at day 20 and progressing out to day 36. Serink et al. found that 1-h daily impact loading of the adult rabbit knee resulted in synovial thickening, cartilage degeneration, and subchondral bone changes which occurred concurrently in joints by 3 weeks [18].
Impact Without Fracture: Animal Models of a Single-Impact Load
Several experiments applied an external impact load across the closed patellofemoral joint at subfracture loads and evaluated the effect on cartilage and subchondral bone. Donohue et al. and Thompson et al. utilized a single impact to the adult canine patellofemoral joint, and found that adult canine articular cartilage showed significant alterations in degenerative histological, structural, and biochemical changes without disruption of the articular surface at 4 and 6 weeks [19], and within 6 months, a single impact resulted in superficial disruption of the cartilage and subchondral changes that lead to arthritic-like degeneration of the cartilage [20]. Chrisman et al. used a subfracture impact load to canine femoral condyles and showed increased arachidonic acid in impacted cartilage [21]. This early study highlighted the association between mechanical injury and early biochemical changes of the arthritic process and the need for better understanding of the biochemical cascade to improve healing. Several others studies were used to characterize the effect of a single impact on joint tissue degeneration using a similar model of impact to the closed patellofemoral joint in skeletally mature rabbits [22–27]. Various factors associated with automobile dashboard-type injuries were investigated, including padding of the impactor, intensity of impact, and rate of impact. In general, a single impact resulted in acute surface fissures of the cartilage, progressive cartilage degeneration and softening by 12 months post-injury, and progressive subchondral bone thickening out to 12 months post-injury with high-intensity impact and no padding.
An important note from this series of investigations was the finding that nonimpact control animals were required to demonstrate statistical significance and that contralateral control limbs were not sufficient for comparisons. These studies model joint damage that may be observed following direct blunt trauma transmitted across articular surfaces without radiographic evidence of fracture. With transarticular loading models, it is difficult to determine the contact area between the articulating surfaces, and therefore difficult to determine the actual impact stress to the cartilage. Pressure films can be inserted between the articular surfaces, but this may interfere with joint congruity and introduce high variability.
Several studies performed assessments following subfracture impact loading of intact patellae to examine the effects of injury on viability of patella articular cartilage as a function of time. When the whole joint capsule is kept intact until testing, and the testing is done on the day of harvest, the articular cartilage is viable and useful to test the effect of injury on viability. Lewis et al. mounted normal bovine patellae and impacted the articular surface with a 6 mm indenter with rounded edges resulting in a subfracture impact of 53 MPa to study cell death as a function of spatial location and time [28]. This level of impact load created cracking in the articular cartilage surface that did not propagate into the underlying subchondral bone. They report that cell death occurred around cracks which were found only in zone 1 (surface layer) or zone 2 of the articular cartilage; with this model cell viability did not decrease with culturing of the cartilage from 18 h to 5 days post-injury, suggesting that in this study with rapid impact loading, the mechanism of cell death was necrosis. The authors reported that chondrocyte viability was affected predominately by cartilage tissue damage (Fig. 8.2). Ashwell et al. impacted porcine patellae between 40 and 50 MPa and examined gene expression immediately after impact and then after organ culture for 14 days [29, 30]. Immediately after impact, mmp3 was upregulated on day 0, and col1a1 was increased at day 14 which suggested that chondrocytes may be more fibroblast-like following impact.
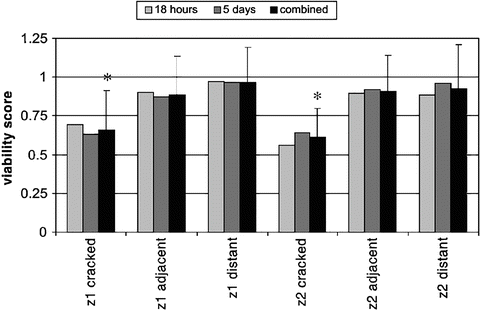
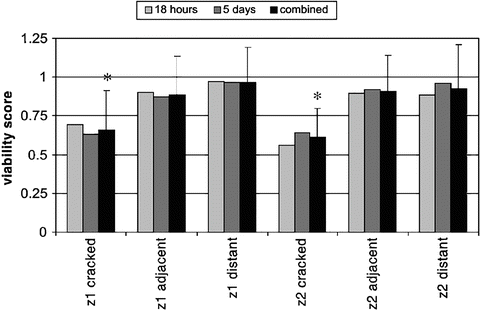
Fig. 8.2
Shows the relationship of chondrocyte viability and location relative to cartilage cracks involving zone 1 or zone II depth. *indicates significantly reduced chondrocyte viability p <.05. Reproduced from Lewis JL et al. Cell death after cartilage impact occurs around matrix cracks JOR 21:881 2003
Recently, Novakofski has demonstrated the feasibility of using high-resolution multiphoton microscopy (MPM) of live, intact cartilage following subfracture impact loading to 30 MPa of equine metacarpal and metatarsal joints [31]. They were able to identify damage to the cartilage and spatial distribution of chondrocyte death using this technique. As previously described by others, cell viability was significantly reduced along the cracks and within the first 100 μm of the superficial layer.
Impact Without Fracture: Animal Models of a Single-Impact Load Through an Arthrotomy
An alternative approach is to surgically open the joint and directly apply a load with an indenter of known surface area. Several studies have used this approach to apply a subfracture impact load to the femoral condyle in a rabbit knee to study the effect of a single traumatic load on cartilage matrix and viability. Milentijevic et al. impacted the lateral femoral condyle of the rabbit knee to 35 MPa and showed matrix damage, proteoglycan loss, and chondrocyte death at 3 weeks post-injury [32]. Borrelli et al. have reported on a range of impact loads (14–100 MPa) to the posterior aspect of the medial condyle in young rabbits at 3 months of age [33–36]. Higher impact loads resulted in acute superficial fissures in the articular cartilage with reported progressive loss of proteoglycan staining in the area of impaction over 6 months. Interestingly, Borrelli et al. reported that loads they had previously established from in vitro studies that resulted in damage in cartilage explants did not translate to in vivo animal model [37]. Higher impact loads were required with intact joints to produce similar levels of cartilage matrix damage and cell death.
Vrahas et al. used cadaver rabbit femoral condyles to develop a drop tower impact model capable of delivering predicable impacts and found that with varying impact energy and indenters, localized cartilage damage to the cartilage and bone was most frequent with a highly localized stress of >17 MPa delivered with a flat indenter [38]. Atkinson et al. found that with low energy impact of 2J, injury to the cartilage was observed, but at high-energy impacts of 22 J, deep injuries to the underlying bone were observed [39]. These studies suggested that the data was applicable to knee trauma occurring during motor vehicle accidents.
Recently, Brophy et al. have incorporated a radial transection of the medial meniscus in the rabbit knee along with the impact loading model developed by Borrelli et al. in young, 3-month-old rabbits [40]. At 3 months post-injury, they found that combined traumatic impact and meniscal transection was more damaging to articular cartilage than meniscal transection alone. Combined injuries to the articular cartilage and meniscus in the knee are common, and these results suggest that clinically combined meniscal and ligament knee injury with significant bone bruising may be more likely to lead to degenerative changes than an isolated meniscal tear.
Animal Models of Articular Fracture
Articular Facture Created with Impact Loading
Whole joint cadaver models of transarticular impact resulting in articular fracture have also been developed. Backus et al. impacted intact porcine knees on the day of joint harvest with a transarticular load via a drop track at high energy of 297 J [41]. With the same impact load, central alignment of the impact load resulted in no fracture, whereas a laterally offset applied impact load resulted in a lateral tibial plateau fracture. As demonstrated by Kennedy, clinically lateral tibial plateau fracture is often created with a valgus stress coupled with an axial load. Osteochondral cores were obtained and cultured up to 5 days postimpact. Chondrocyte death, cartilage proteolytic enzyme activity, and S-GAG release were significantly upregulated in impacted joints that sustained an articular fracture. Increased levels of chondrocyte death were not observed in those joints that were impacted without creation of a fracture. Similar to data from Lewis et al., chondrocyte viability was not significantly affected by culture time post-trauma.
Tochigi et al. also impacted porcine hocks via a drop track (30 J) with introduction of a saw cut defect to the distal tibial cortex to mimic human ankle fractures, and cell viability of impacted fractured joints was compared to fractures created via an osteotomy [42]. Impacted fractured joints demonstrated ninefold greater chondrocyte death along the fracture edge compared to osteotomy-induced fractured joints. The authors concluded that the model development and data supported its translation to an in vivo study with survival animal surgeries.
Models of Articular Fracture Created with Osteotomy
A group of animal studies of intra-articular fracture (IAF) have mainly focused on cartilage healing with and without anatomic reduction of the articular surface. Mitchell and Shepard used an osteotomy model in a rabbit knee to study healing following a fracture-like disruption of the cartilage and subchondral bone. They observed hyaline-like cartilage healing following interfragmentary compression of the disrupted cartilage and bone [43]. Several other animal studies demonstrated, again with osteotomy models of articular fracture, that restoration of the subchondral plate and articular surface was possible even with incongruences of the articular cartilage [44–48]. However, healing was also associated with the development of osteophytes and other signs of degenerative arthritis. These studies indicate that some degree of articular surface remodeling is possible, but the progression of osteoarthritic changes with remodeling is unknown. Additionally, all of these studies utilized open surgical models that evacuate the hemarthrosis and do not incorporate blunt trauma to the articular surface and synovium. Clinically, these components of articular fracture cannot be isolated.
In Vivo Animal Models of Articular Fracture with Impact
Larger Animal Models
Animal models of articular fracture that incorporate both disruption of the articular cartilage and subchondral bone with articular impact may provide the most insight into the mechanisms of PTA development. The few in vivo articular models of articular fracture with impact are summarized here. In 1975, Farkas et al. created articular fractures in the femoral condyles of rabbits and reported that repair of the articular fracture was observed by 6 weeks postfracture with normal cartilage structure but a fibrous overgrowth of tissue that resembled a synovial pannus [49]. They concluded that no irreversible damage in cartilage composition was found with articular fracture in the rabbit knee at 8 weeks postfracture.
Another closed joint fracture model has been developed in the rat knee. A drop tower applied an impact to the closed patellofemoral joint of the rat knee to create intercondylar femoral fractures, again similar to a dashboard-type injury associated with automobile accidents. Chondrocyte viability was reported to drop from 68 % at day 0 to 46 % at 72 h following fracture. The level of cell death following fracture is similar to previous reports from cadaveric studies of impact with articular fracture.
Diestelmeirer et al. reported recently on the development of an instrumented pendulum impaction system to generate fractures of the distal tibia in mini-pigs to model human tibial pilon fractures [50]. As reported in the model development with cadaver animals, fractures were generated with introduction of a saw cut defect to the distal tibial cortex. Although change to joint tissues was not yet reported, fractures exhibited the general clinical appearance of human tibial pilon fractures and energy absorbed during fracture was reported.
Olson et al. developed a dorsal wall fracture of the acetabulum in the goat hip [7]. In order to generate articular fractures, stress risers were surgically introduced in the posterior wall by predrilling and scoring the retroacetabular surface of posterior wall. The fractures were then generated with an impact (40–60 MPa) from a drop tower applied at the flexed knee in line with the femoral shaft resulting in a transarticular impact to the hip, similar to dashboard injury. Joints were then assessed with and without anatomic reduction at 90 days post-surgery. The residual defects were observed to be filled with fibrous tissue. Fractured joints demonstrated greater histologic scores of arthritis than control hips, and scores trended towards being greater in the displaced group. Such large animal models in mini-pigs or goats can provide a means to study the effects of treatment of displaced IAF.
The use of closed-joint articular fracture in a large animal model will allow investigation of joint injury treated with surgical realignment and fixation. Creating the fracture without arthrotomy, or opening of joint capsule, may allow for a more clinically relevant evaluation of the sequelae of joint degeneration following trauma to the cartilage and subchondral bone without the potentially confounding elements of surgical disruption of the capsule and synovium. Increasingly, survival models of articular fracture are leading to important contributions in the mechanisms of PTA development. However, use of a larger animal model in preliminary investigations attempting to understand basic mechanisms of joint injury is cost prohibitive.
Murine Model of Intra-articular Fracture
Murine models have the advantage of a relatively low cost of experimental animals; well-described analyses for serum and synovial biomarkers; and transgenic strains of mice are readily available. A murine model of IAF may provide insight into basic mechanisms of post-joint injury response that lead to PTA. Success in identifying the mechanisms involved in the progression of PTA after fracture could directly impact clinical practice in treating articular fractures.
One of the benefits of collaboration between clinicians and basic scientists is the opportunity for synergy in approach to research. The development of a murine model of closed IAF is the result of such synergy. The initial challenge was the lack of a validated model of an IAF that developed PTA. Surgeons interested in studying articular fractures often focus on the importance and effects of realigning or reduction of the articular surface as part of the treatment of the injury. Basic scientists seek to understand basic mechanisms in play leading to the outcome of interest. The collaboration of these two perspective results in the development of a novel murine model of a closed IAF that develops PTA to observe the natural history of development of PTA after an IAF.
Furman et al. described the mouse model of closed articular fracture of the tibial plateau for studying PTA (Fig. 8.3). The articular fractures are generated using a computer-controlled material testing system and custom-built indenter tip to apply a load to the anterior aspect of articular cartilage of tibial plateau. A detailed description is found in the original manuscript [51]. The indenter is applied in a load control mode that allows for creation of high-energy and low-energy articular fractures. The energy of injury is measured as the energy applied at the time of fracture creation. In order to validate this simple measure we performed an analysis determining the liberated area after an articular fracture using micro CT images of the fractured limb and the contralateral normal limb as described by Beardsly et al. There was a strong correlation between measured energy of fracture and liberated area (R 2 = 0.70) (Fig. 8.4).
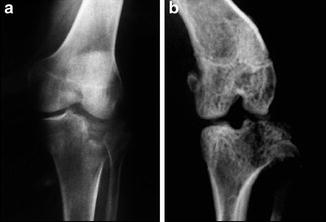
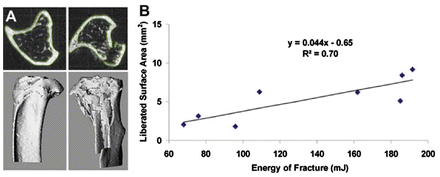
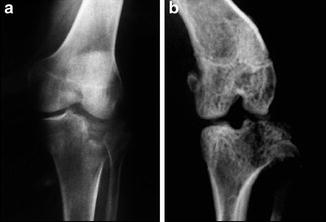
Fig. 8.3
Radiographs of a tibial plateau fracture in a human knee (a) and a mouse knee (b) (reproduced from Furman et al. Joint Degeneration following Closed Intra-articular Fracture in the Mouse Knee: A Model of Post-Traumatic Arthritis J Ortho Res 25:578, 2007)
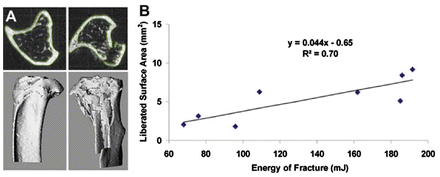
Fig. 8.4
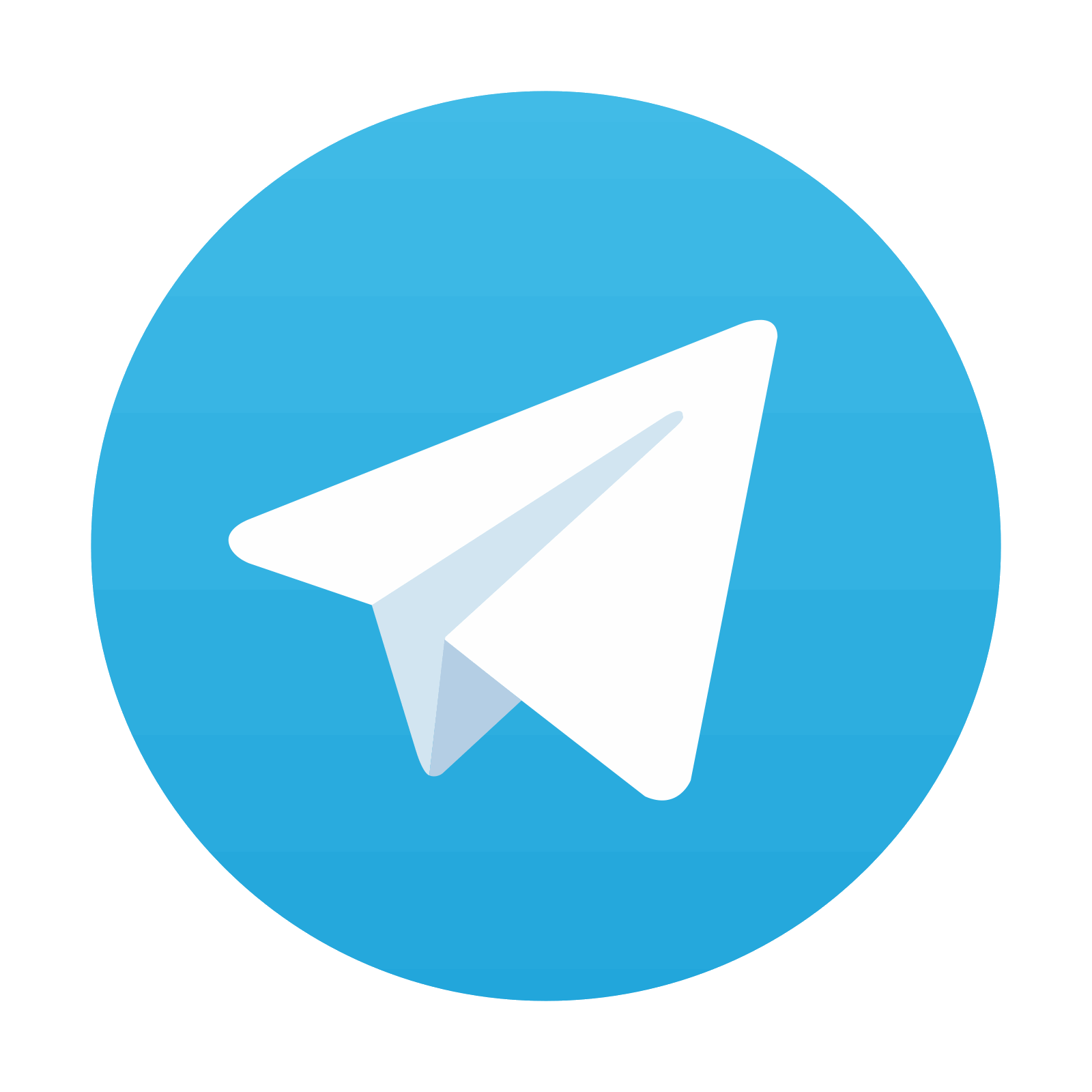
(a) Illustrates the technique of peripheral surface measurement used to determine the exposed surface area for the intact and fractured limbs. The difference between the exposed surface area of the two sides gives the surface area liberated by the articular fracture. (b) The correlation between liberated surface area and measured energy of fracture is shown (reproduced from Lewis et al. Acute joint pathology and synovial inflammation is associated with increased intra-articular fracture severity in the mouse knee. OA & C 19:864, 2011)
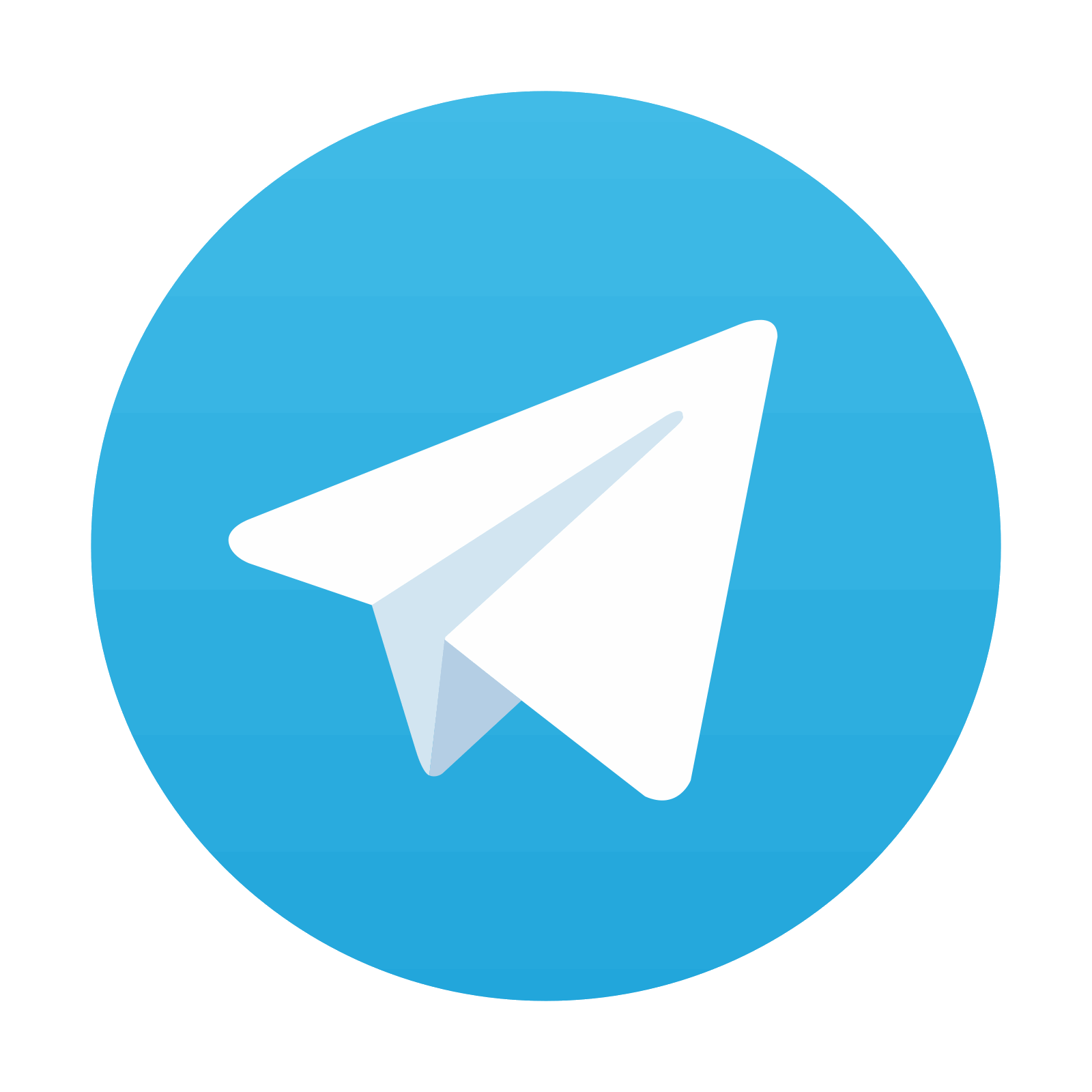
Stay updated, free articles. Join our Telegram channel
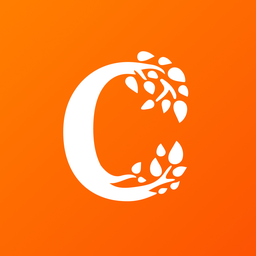
Full access? Get Clinical Tree
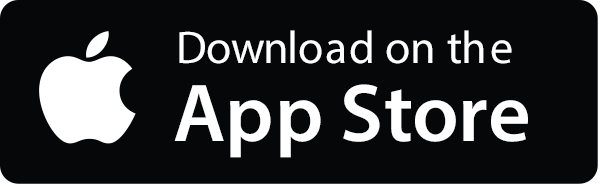
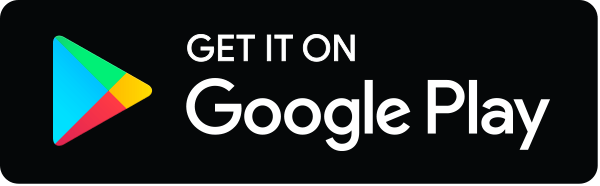
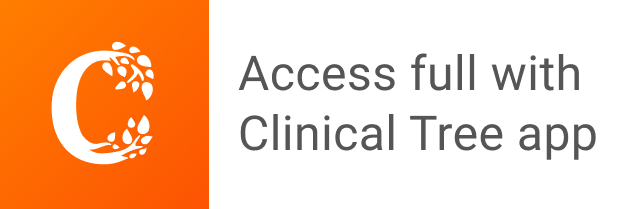