Fig. 9.1
An example of a video matched in Poser, three-camera basketball injury situation 50 ms after IC. The two top panels and left bottom panel show the customized skeleton model and the basketball court model superimposed on and matched with the background video image from three cameras with different angles. The right bottom two panels show the skeleton model from a side view created in Poser
The knee kinematical patterns were remarkably consistent among the ten cases (Fig. 9.2). The knee was relatively straight, with a flexion angle of 23° (range, 11–30°), at initial contact (IC) and had increased by 24° (95 % CI, 19–29°, p < 0.001) 40 ms later. The knee abduction angle was neutral, 0° (range, −2° to 3°) at IC, but had increased by 12° (95 % CI, 10–13°, p < 0.001) 40 ms later. As for knee rotation angle, the knee was externally rotated 5° (range, −5° to 12°) at IC, but abruptly rotated internally by 8° (95 % CI, 2–14°, p = 0.037) during the first 40 ms. From 40 ms to 300 ms after IC, however, we observed an external rotation of 17° (95 % CI, 13–22°, p < 0.001). In addition, the estimated peak vertical ground reaction force (GRF) was 3.2 times body weight (95 % CI, 2.7–3.7), and occurred at 40 ms (range, 0–83) after IC. On the other hand, the hip kinematics was relatively constant at a 20° abducted, 50° flexed and 30° IR position during 40 ms after IC (Fig. 9.3).
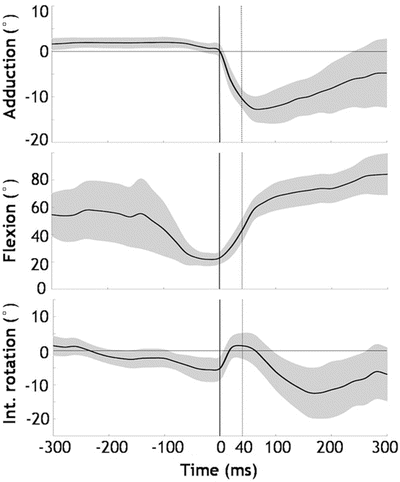
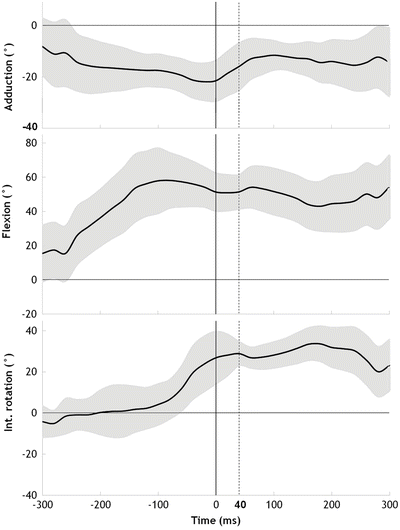
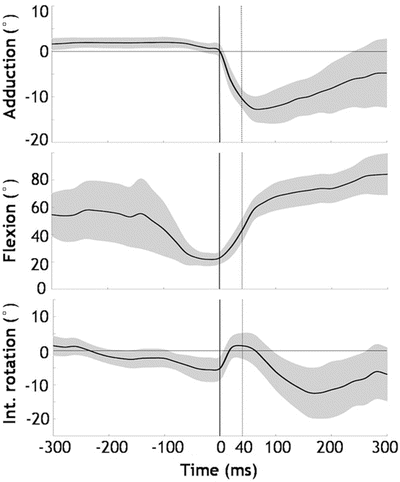
Fig. 9.2
Time sequences of the mean knee angles (°) (black line) of the 10 cases with 95 % confidence intervals (CI) (grey area). Time 0 indicates IC and the dotted vertical line indicates the time point 40 ms after IC
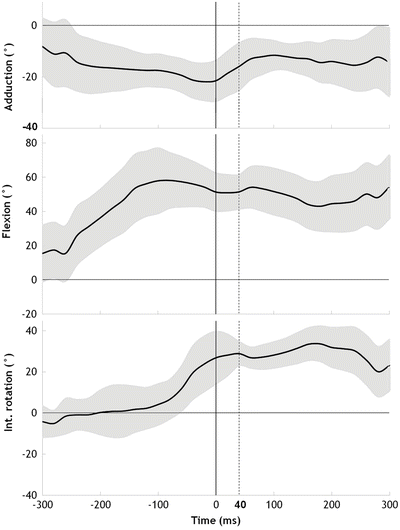
Fig. 9.3
Time sequences of the mean hip angles (°) (black line) of the 10 cases with 95 % CI (grey area). Time 0 indicates IC and the dotted vertical line indicates the time point 40 ms after IC
However, a limitation of the above mentioned analysis was how accurate the joint kinematics and timing of peak GRF could be estimated from the relatively low frame rate (50 or 60 Hz) and low quality images (768 × 576 pixels) in analog video sequences, and therefore we were unable to assess the anterior translation of the tibia. However, a noncontact ACL injury situation in a male footballer was available which had been recorded using four high-definition (HD, 1080i) cameras, including two high-speed recordings (100 and 300 Hz). In this case, the 26-year old male elite football player suffered a noncontact ACL injury to his right knee during a national team match, when he tried to stop after having passed the ball with his right leg. This case was analyzed using the MBIM technique to describe the more detailed joint kinematics, including tibial translations (Fig. 9.4). Knee kinematics in this case were strikingly consistent with the previous analyses of the ten cases (Fig. 9.5). The knee was flexed 35° at IC, with initial extension (26° of flexion) until 20 ms after IC, after which flexion angle continued to increase. The knee abduction angle was neutral at IC, but had increased by 21° 30 ms later. The knee was externally rotated 11° at IC, but abruptly rotated internally by 21° during the first 30 ms, then changed its direction to external rotation after this. In addition, anterior tibial translation was able to be detected; it started to occur at 20 ms after IC, where the knee was the most extended, and by 30 ms after IC approximately 9 mm of anterior translation had occurred. The translations plateaued by 150 ms, and then shifted back to a reduced position between 200 ms and 240 ms after IC.
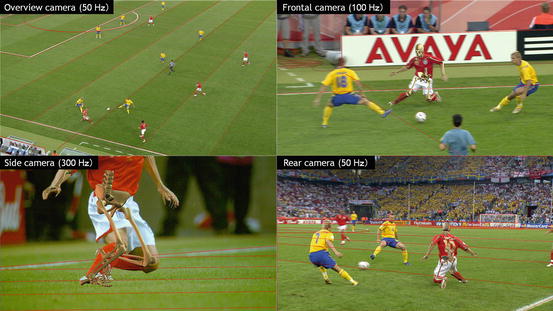
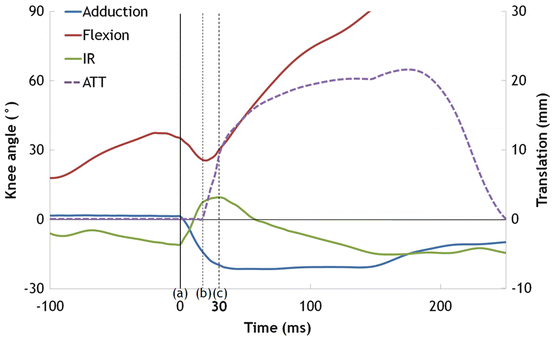
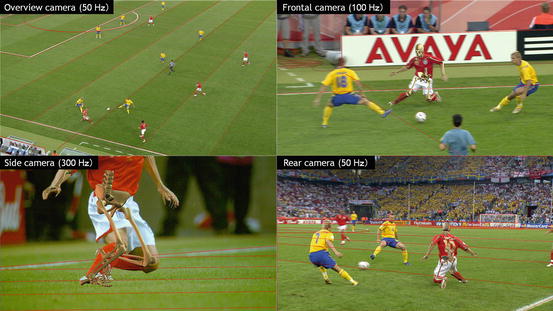
Fig. 9.4
A soccer injury situation recorded using HD cameras. Each panel shows the customized skeleton model and the football pitch model superimposed on and matched with the background video image from each camera. Overview camera and rear camera had an effective frame rate after being deinterlaced of 50 Hz, frontal camera 100 Hz and side camera 300 Hz
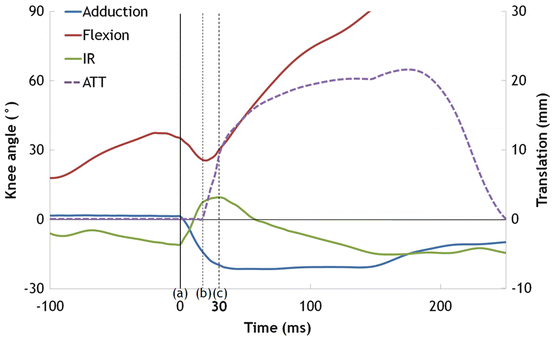
Fig. 9.5
Time sequences of knee joint angles (left axis) and anterior tibial translation (right axis) in the soccer case. Time 0 (a) indicates IC and the dotted vertical lines (b) and (c) indicate the time point 20 and 30 ms after IC, respectively
9.3 Timing of Non-contact ACL Injury
It has not been possible to determine the exact timing of ACL injury from video analysis based on simple visual inspection (Boden et al. 2000; Krosshaug et al. 2007a; Olsen et al. 2004). However, this may be possible by using the MBIM technique, by assessing abnormal joint configurations, sudden changes in joint angular motion and timing of GRFs. The extracted knee kinematics during ACL injuries using the MBIM technique showed that sudden increase of valgus and internal rotation angle occurred within the first 40 ms after IC. These periods also correspond to the average peak vertical GRF in these cases. Moreover, in the case recorded using HD cameras, abrupt anterior tibial translation reached 9 mm in 30 ms after IC, which corresponds to the maximum anterior translation in intact knees (Jakob et al. 1987; Meyer and Haut 2008). Based on these results, together with the previous studies showing that the ACL was strained shortly (approximately 40 ms) after IC in simulated landing (Withrow et al. 2006; Shin et al. 2007), it seems likely that the injury occurs within 40 ms for the majority of these cases.
9.4 Mechanism for Non-contact ACL Injury
As already mentioned, valgus collapse in combination with external rotation (i.e. knee in, toe out) has frequently been identified as an ACL injury mechanism based on simple visual inspection of injury video tapes. However, it has been discussed as to whether these kinematics actually represent the cause for ACL injuries or simply are a result of the ACL being torn (Olsen et al. 2004; Ebstrup and Bojsen-Moller 2000). Our results using the MBIM technique showed that immediate valgus motion occurred within 40 ms after IC. The abrupt internal rotation also occurred during the first 40 ms after IC, then external rotation was observed, which seems to have occurred after the ACL was torn. In addition, anterior tibial translation started a little after IC, and increased abruptly until when the injury might have occurred. The discrepancy between the previous studies and our results could be that the abrupt internal rotation and anterior tibial translation observed using the MBIM technique analysis are likely not easily detected from visual inspection alone; the external rotation that occurs afterwards is more pronounced and therefore easier to observe. The internal-to-external rotation sequence with anterior tibial translation has also been reported previously. In a recent cadaver study, the application of pure compressive loads led to anterior tibial translation and internal tibial rotation of up to 8°, followed by a sudden external rotation of 12° (Meyer and Haut 2008). The combination of internal tibial rotation and anterior tibial translation is probably caused by the joint surface geometry. The concave geometry of the medial tibia facet combined with the slightly convex lateral tibia facet may cause the lateral femoral condyle to slip back. This may also explain why ACL-injured patients tend to have greater posterior lateral tibial plateau slopes than uninjured controls (Brandon et al. 2006; Stijak et al. 2008; Hashemi et al. 2010).
Combining the results obtained using the MBIM technique with previous findings, the following hypothesis for the mechanism of non-contact ACL injury is proposed (Fig. 9.6) (Oiestad et al. 2009): when valgus loading is applied, the MCL becomes taut and lateral compression occurs (Caraffa et al. 1996). This compressive load causes a lateral femoral posterior displacement, probably due to the posterior slope of lateral tibial plateau, and the tibia translates anteriorly and rotates internally, resulting in ACL rupture (Gilchrist et al. 2008). After the ACL is torn, the primary restraint to anterior translation of the tibia is gone. This causes the medial femoral condyle to also be displaced posteriorly, resulting in external rotation of the tibia. This external rotation may be exacerbated by the typical movement pattern when athletes plant and cut, where the foot typically rotates externally relative to the trunk.
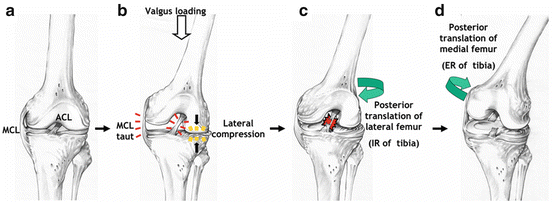
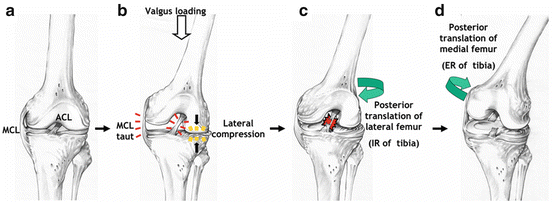
Fig. 9.6
The proposed non-contact ACL injury mechanism. (a) An unloaded knee. (b) When valgus loading is applied, the MCL becomes taut and lateral compression occurs. (c) This compressive load causes a lateral femoral posterior displacement, probably due to the posterior slope of lateral tibial plateau, and the tibia translates anteriorly and rotates internally, resulting in ACL rupture. (d) After the ACL is torn, the primary restraint to anterior translation of the tibia is gone. This causes the medial femoral condyle to also be displaced posteriorly, resulting in external rotation of the tibia
9.5 The Role of the Hip in Preventing ACL Injury
The lower extremities act as a kinetic chain during dynamic tasks and the control of hip motion substantially affects the knee motion. Researchers have studied the relationships between hip biomechanics and ACL injury. As for hip biomechanics being a risk factor for ACL injury, Decker et al. (2003) reported that, in drop landing, energy absorption at the hip joint, and hip flexion angles at IC were less in females than in males. Schmitz et al. (2007) reported that, in single-leg landing, energy absorption at the hip and total hip flexion displacement were smaller in females, whereas peak vertical GRF was larger in females. Yu et al. (2006) also reported that hip flexion angular velocity at IC was negatively correlated with peak vertical GRF in a stop-jump task. When it comes to ACL injury mechanisms, Heshemi et al. (2007) reported that, in a cadaver study, a restricted flexion of the hip at 20° combined with low quadriceps and hamstrings force levels in simulated single-leg landing were found to be conducive to ACL injury. A video analysis has shown that ACL injured subjects’ hip flexion and abduction angles were constant during 100 ms after IC, whereas uninjured control subjects’ hip flexion increased by 15° in cutting/landing maneuvers (Boden et al. 2009). Our study using MBIM technique also showed that hip kinematics was constant during 40 ms after IC in an abducted, flexed and internally-rotated position, which seems to play a significant role in the mechanism of ACL injury. In this regard, Hashemi et al. (2011) have proposed a mechanism called “hip extension, knee flexion paradox”, i.e. that a mismatch between hip and knee flexion in landing is the cause of ACL injury. In normal conditions, both the knee and the hip flex together in landings, whereas in unbalanced landings, the knee is forced to flex while the hip is forced to extend, and the tibia will undergo anterior translation, which will increase the risk of ACL injury.
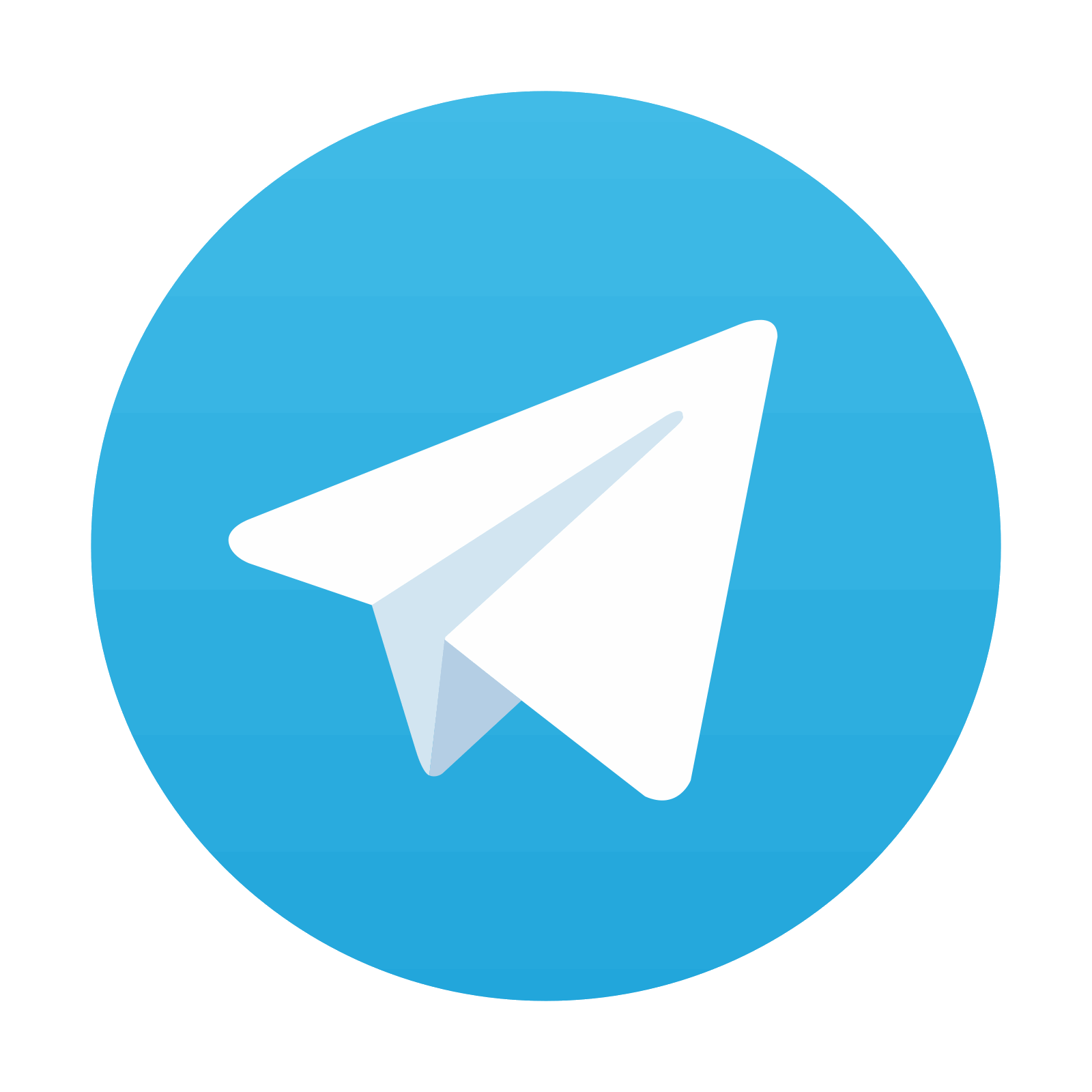
Stay updated, free articles. Join our Telegram channel
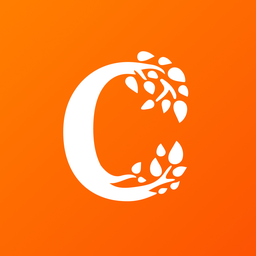
Full access? Get Clinical Tree
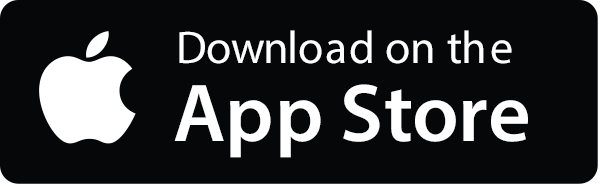
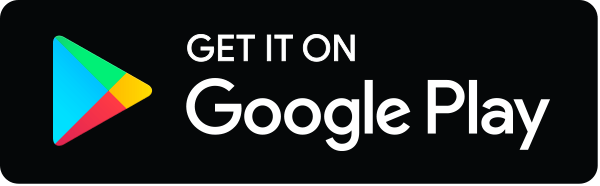