Ventilation and Oxygenation
Donald A. Reiff
L. Christopher DeRosier
Loring W. Rue III
HISTORY
Mechanical ventilation was first described by Versalius during the 16th century. He conducted experiments by inserting a reed into the trachea of animals and then blowing into the conduit, demonstrated adequate ventilation. The first known device constructed expressly to provide mechanical ventilation was created by Fell O’Dwyer in 1888. The basic design was similar to an accordion that would be intermittently compressed delivering a volume of gas to the patient. During the following 90 years, ventilators have become technically more sophisticated; however, volume-cycled ventilation remains the principal mode by which mechanical ventilation is delivered. Alternate advanced modes of mechanical ventilation became available during the early 1980s when technologically complex microprocessors were incorporated into the design of mechanical ventilators. The addition of minicomputers to ventilators has broadly expanded the capabilities of our invasive monitoring of the pulmonary cycle enabling a more accurate measurement of airway pressures and comprehensive assessment of pulmonary compliance. While no data exists to support the fact that these sophisticated microprocessor ventilators achieve significantly improved results above those rendered by conventional ventilators, microprocessor-controlled ventilators have several intuitive advantages in the management of the critically ill patient. In addition to a more compact design, microprocessor-driven ventilators possess the added advantages of enhanced monitoring capabilities, computer correction capabilities, improved and comprehensive liquid crystal display (LCD) display, and the addition of several new spontaneous and mechanical modes of ventilation not previously available.
RESPIRATORY PHYSIOLOGY
Ventilation is defined as the movement of air from the exterior environment to the alveolus. Although the principal function of the lung is gas exchange within the alveolus, ventilation is solely descriptive of the mechanical function of the lung. Inadequate ventilation due to any number of factors is often an underappreciated cause of respiratory dysfunction and significant morbidity among injured and postoperative patients.
When considering the mechanical process of ventilation, it is helpful to define and understand pulmonary volumes and capacities used to describe all phases of the respiratory cycle (see Fig. 1). Tidal volume (VT) describes the volume of air inspired and expired in a normal breath. The extra volume of air that can be inspired beyond a normal VT is termed inspiratory reserve volume (IRV). Similarly, at the end of a normal VT the additional volume of air available to exhale is the expiratory reserve volume (ERV). The volume of air remaining within the lungs following maximal expiration is referred to as the residual volume (RV). The critical closing volume (CCV) represents the volume of air required within the pulmonary tree necessary to maintain the patency of small airways.
By combining one or more pulmonary volumes, the four pulmonary capacities are established. Functional residual capacity (FRC) represents the amount of air remaining within the lung following normal expiration and is the sum of the ERV and RV. The sum of the IRV, VT, and ERV is termed the vital capacity (VC), or the maximal amount of expired air after deepest inspiration. Total lung capacity (TLC) is the greatest volume to which the lungs can be expanded and is formed as the sum of the VC and the RV.
The maximal volume of air that can be inspired following a normal expiration is termed the inspiratory capacity (IC) and is determined by the sum of VT and IRV. The ability to cycle volumes of air during the phases of respiration is affected by several factors including the mechanical resistance of chest wall and abdomen, airway resistance, and pulmonary compliance of the expanding lung parenchyma.
The maximal volume of air that can be inspired following a normal expiration is termed the inspiratory capacity (IC) and is determined by the sum of VT and IRV. The ability to cycle volumes of air during the phases of respiration is affected by several factors including the mechanical resistance of chest wall and abdomen, airway resistance, and pulmonary compliance of the expanding lung parenchyma.
![]() Figure 1 Diagram depicting ventilation excursion during normal respiration and maximum effort of inspiration and expiration. (Courtesy of Anesthesia UK.) |
Airway resistance is the opposition to flow caused by the forces of friction. It is defined as the ratio of driving pressure to the rate of airflow. Resistance to flow in the airways depends on whether the flow is laminar or turbulent, on the dimensions of the airway, and on the viscosity of the gas. The viscosity of gases used during conventional mechanical ventilation remain relatively constant whereas the other two variables are quite dynamic among patients within the intensive care unit (ICU).
Laminar flow resistance is calculated using the Poiseuille Law: R = 81η/πr4 where resistance is directly proportional to the length of the conduit and inversely related to the radius of the tubing raised to the fourth power. Therefore, very subtle changes in the radius of the ventilator circuit, particularly the endotracheal tube, will have rather profound effects on the airflow. Whereas the resistance to flow is quite low under laminar conditions such that minute changes in driving pressures will result in substantial changes in flow rates, under turbulent conditions resistance is relatively large necessitating much higher driving pressures for the same flow rate. Common conditions resulting in disturbance of laminar flow include partial obstruction of the endotracheal tube by inspissated secretions, bronchospasm, endotracheal tube abutting the carina and fluid trapped within the ventilator circuit tubing.
Airway resistance decreases throughout inspiration as a consequence of increasing airway distension, wider airways provide less resistance (see Fig. 2). Furthermore, although a single small airway provides more resistance than a single large airway, resistance to airflow also depends on the number of parallel pathways present. That is, airway resistance initially increases as air travels from the single large trachea to the smaller mainstem bronchi and onward to lobar bronchi. However, as the airways continue to bifurcate, the overall surface area of the channels exponentially expands resulting in drastic reduction in airway resistance (see Fig. 3). Subtle changes in airway diameter at the terminal bronchiolar level, as seen in patients with reactive airway disease, will have profound adverse effect on airway resistance.
A second important factor affecting ventilation is abdominal and chest wall elastic resistance. Increased thoracic stiffness can be caused by chest wall edema, contusion, hematoma associated with rib fractures, circumferential escar formation associated with severe truncal burn wounds,
morbid obesity and/or thoracic spine fractures. Irrespective of the cause, any reduction in elasticity of the chest wall is correspondingly associated with worsening compliance and loss of ventilation capacity. Among patients sustaining blunt force thoracoabdominal trauma, rib fractures and chest wall contusion is the most common etiology of worsening elastic resistance. The loss of ventilatory capacity as a consequence of four rib fractures has been associated with substantial morbidity and mortality.1
morbid obesity and/or thoracic spine fractures. Irrespective of the cause, any reduction in elasticity of the chest wall is correspondingly associated with worsening compliance and loss of ventilation capacity. Among patients sustaining blunt force thoracoabdominal trauma, rib fractures and chest wall contusion is the most common etiology of worsening elastic resistance. The loss of ventilatory capacity as a consequence of four rib fractures has been associated with substantial morbidity and mortality.1
![]() Figure 2 Increases in pulmonary volume result in airway distension and a corresponding fall in airway resistance. (Ball WC. Johns Hopkins School of Medicine’s Interactive Respiratory Physiology.) |
The most frequent factor associated with reduction in thoracic and diaphragmatic elasticity among elective surgical patients is abdominal surgery. As a consequence of the laparotomy, intestinal and retroperitoneal edema coupled with bowel distension results in increased abdominal volume. The bony pelvis and strong musculature laterally limit the expansion of the abdominal cavity inferiorly and laterally, the increased abdominal volume is therefore limited to anterior and cephalad expansion. If the rectus and oblique muscles relax, anterior abdominal distension results allowing for downward diaphragmatic excursion and improved ventilation. If however, the abdominal musculature is contracted in response to somatic pain, the diaphragm and anterior abdominal musculature are in direct opposition to one and other during all phases ventilation. The greater strength of the rectus and oblique muscles will overcome the diaphragm, causing cephalad displacement of the abdominal contents toward the thoracic cavity impairing effective ventilation.
Irrespective of whether a patient has sustained trauma or is recovering from elective surgery, VC is reduced in the immediate postinjury period.2,3 The loss of VC associated with elective abdominal surgery can routinely approach 50%; this coupled with abdominal distension directed toward the thorax can impede ventilation by as much as 70%. Although the loss of VC is significant, FRC losses are critical. Ventilation capacity loss associated with reduction in FRC is underscored by the concept of CCV. Under normal conditions, the FRC exceeds the CCV and small airways remain open. Elective thoracic and/or abdominal surgery, chest wall trauma, interstitial edema, recumbent position, and acute respiratory deficiency syndrome (ARDS) are medical/surgical conditions that can lead to a reduction in FRC below CCV causing progressive bronchiolar and alveolar collapse, with significant atelectasis resulting in profound alterations in gas exchange and exacerbating the risk of pulmonary infectious complications. The detrimental effect of the lost FRC can be negated and atelectatic lung reclaimed by delivery of positive end-expiratory pressure (PEEP), which enables the rerecruitment of these collapsed alveoli.4,5,6
Under normal circumstances, the elastic recoil of the lung should be considered in conjunction with the chest wall. Although they are mechanically coupled and function inseparably, a number of pathophysiologic conditions can lead to altered pulmonary compliance without an alteration in chest wall elasticity. Pulmonary fibrosis, ARDS, pulmonary hypertension, and pulmonary edema will substantially reduce pulmonary elasticity while chest wall compliance remains relatively unaffected. Pulmonary resistance may exceed that of the chest wall by several fold.7
Pulmonary compliance is defined as the ratio of change in lung volume to change in pulmonary pressure (ΔV/ΔP) and is used to represent the relative stiffness of the pulmonary parenchyma and chest wall. Most modern ventilators now enable physicians to easily and accurately calculate this value at the bedside on a real-time basis thereby permitting changes in ventilator strategies to maximize lung function while limiting barotrauma. When considering pulmonary compliance, it is helpful to quantitate the value without the effect of airflow through the pulmonary conduits, which is defined as static compliance (Cstat). During inspiration, pressure is exerted on the pulmonary parenchyma by gases moving through the airways, thereby raising the value of the denominator in the calculation of compliance. When attempting to minimize the barotrauma induced by mechanical ventilation and assess the true elasticity of the alveolar unit, the effect of moving gases within the airway should be eliminated. This is accomplished by measuring the airway pressure at the conclusion of inspiration by performing an inspiratory pause to yield the plateau pressure. The set PEEP is subtracted from the end inspiratory pause pressure, or plateau pressure, for the calculation of the Cstat represented in the formula: Cstat = VT/(plateau pressure – PEEP). The pulmonary peak pressure is measured at the end of inspiration and is used to calculate the dynamic compliance (Cdyn) wherein Cdyn = VT/(peak pressure – PEEP).
The importance of minimizing high pulmonary pressures throughout the respiratory cycle cannot be overemphasized. Several landmark studies have demonstrated
significant survival advantage and reduction in morbidity among patients in whom the peak and plateau pressures were restricted below 25 cm H2O.8 Furthermore, other investigators have demonstrated that acute lung injury (ALI) may be induced in patients with physiologically normal lung parenchyma who are ventilated with excessively high VTS (>8 mL per kg ideal body weight).9,10 Current data suggests that volume-restricted and pressure-limited ventilation is superior. VTS are recommended not to exceed 6 mL per kg ideal body weight with peak pressure limited to <25 cm H20.8,11
significant survival advantage and reduction in morbidity among patients in whom the peak and plateau pressures were restricted below 25 cm H2O.8 Furthermore, other investigators have demonstrated that acute lung injury (ALI) may be induced in patients with physiologically normal lung parenchyma who are ventilated with excessively high VTS (>8 mL per kg ideal body weight).9,10 Current data suggests that volume-restricted and pressure-limited ventilation is superior. VTS are recommended not to exceed 6 mL per kg ideal body weight with peak pressure limited to <25 cm H20.8,11
Differences in peak and plateau pressures can be used to evaluate acute deterioration in respiratory function among mechanically ventilated patients. An acute increase in peak pulmonary pressure with concomitant increase in plateau pressure is found among patients with worsening pulmonary compliance. The worsening compliance could result from any number of factors including abdominal distension associated with abdominal compartment syndrome, dysynchronous breathing against the mechanical ventilator, atelectasis and/or lobar collapse, auto-PEEP, ARDS, and pneumothorax. Mechanically ventilated patients with stable plateau pressures in whom peak pressures are progressively increasing should be evaluated for airway obstruction due to aspiration, inspissated pulmonary secretions, bronchospasm, or mainstem bronchial intubation.
Among patients without pulmonary injury and normal lung elasticity, normal Cstat values will range from 50 mL per cm H2O to 80 mL per cm H2O. As the degree of pulmonary insult worsens as a consequence of trauma and/or inflammatory changes associated with injury, compliance values will fall to the range of 20 mL per cm H2O to 50 mL per cm H2O. When compliance is found <40 mL per cm H2O, ventilation independent of mechanical assistance becomes impossible even among the young and healthy. ARDS is hallmarked by diffuse pulmonary infiltrates, severe hypoxemia (PaO2/FIO2 ratio <200), noncardiogenic pulmonary edema, and extraordinarily poor compliance measured <20 mL per cm H2O. Several studies have documented compliance, and sometimes more importantly changes in compliance, can be used as a predictor of mortality among ICU patients.12,13
While establishing an orotracheal or surgical airway should not be taken lightly, instituting mechanical ventilation should not be viewed as failure. All too often, postoperative and injured patients will exert increased work of breathing, worsening hypoxemia, atelectasis, and lobar collapse with acute pulmonary failure whereas the inexperienced introduce often-ineffective measures to improve pulmonary physiology in an effort to avoid orotracheal intubation. The efficacy of noninvasive ventilation (NIV) among a wide spectrum of patient populations including those with chronic obstructive pulmonary disease (COPD) exacerbation, reactive airway disease, obstructive sleep apnea, and cardiogenic pulmonary edema is well established.14,15,16 While exacerbation of many chronic medical conditions are well managed by NIV, the role for noninvasive assistance with ventilation for the acutely injured is less well established and somewhat controversial.17,18,19
MECHANICAL VENTILATION
Continuous Positive Airway Pressure/Bilevel Positive Airway Pressure
Continuous positive airway pressure (CPAP) ventilation and bilevel positive airway pressure (BiPAP) ventilation are similar methods of augmenting the patient’s own ventilatory efforts through a tight fitting nasal or facial mask. CPAP ventilation delivers continuous positive airway pressure (usually 5-10 cm H2O) throughout the respiratory cycle, which aids in increasing FRC and Pao2 levels with the expansion of atelectatic alveoli. BiPAP provides two different levels of positive pressure. A higher pressure (usually 10 cm H2O) is delivered during inspiration (inspiratory airway pressure [IPAP]) whereas a lower pressure (usually 5 cm H2O) is delivered during expiration (expiratory airway pressure [EPAP]) and functions as PEEP in patients with a secure nose/mouth mask. Patients who demonstrate the greatest benefit from noninvasive forms of ventilation are those suffering from COPD exacerbations.20 Contraindications to these noninvasive forms of ventilation include decreased mental status, suspected facial or airway trauma, suspected gastrointestinal trauma, untreated pneumothorax, and hypotension. Although studies have shown some indications and advantages of noninvasive positive airway ventilation in avoidance of intubation,21 this does not apply to the multitrauma patient who requires oxygenation and/or ventilation support. It is the practice of our institution to avoid noninvasive modes of ventilation in the acute setting following traumatic injury and for patients with multisystem traumatic injury who demonstrate pulmonary deterioration during their hospitalization. If the decision is made to use noninvasive positive pressure ventilation, a functioning nasogastric tube as well as a tube thoracostomy (if pneumothorax is suspected) is essential in preventing aspiration or tension pneumothorax respectively.
CPAP and BiPAP are tempting alternatives in patients who have recently been extubated and who have deteriorating oxygenation so as to potentially avoid reintubation. A large multicenter randomized clinical trial of extubated patients who demonstrated postextubation respiratory insufficiency compared standard medical therapy to medical therapy that included NIV. They concluded that although NIV did not reduce mortality or need for reintubation, there was a trend to higher mortality rates among those assigned to NIV.22 Although only a small number of these patients were trauma patients (10% in the NIV group and 7% in the standard medical therapy group), it is reasonable to
extrapolate this data to the trauma patient and not rely on CPAP or BiPAP as a mode of avoidance of reintubation in the recently extubated trauma patient with postextubation respiratory insufficiency.
extrapolate this data to the trauma patient and not rely on CPAP or BiPAP as a mode of avoidance of reintubation in the recently extubated trauma patient with postextubation respiratory insufficiency.
Endotracheal Intubation and Modes of Ventilation
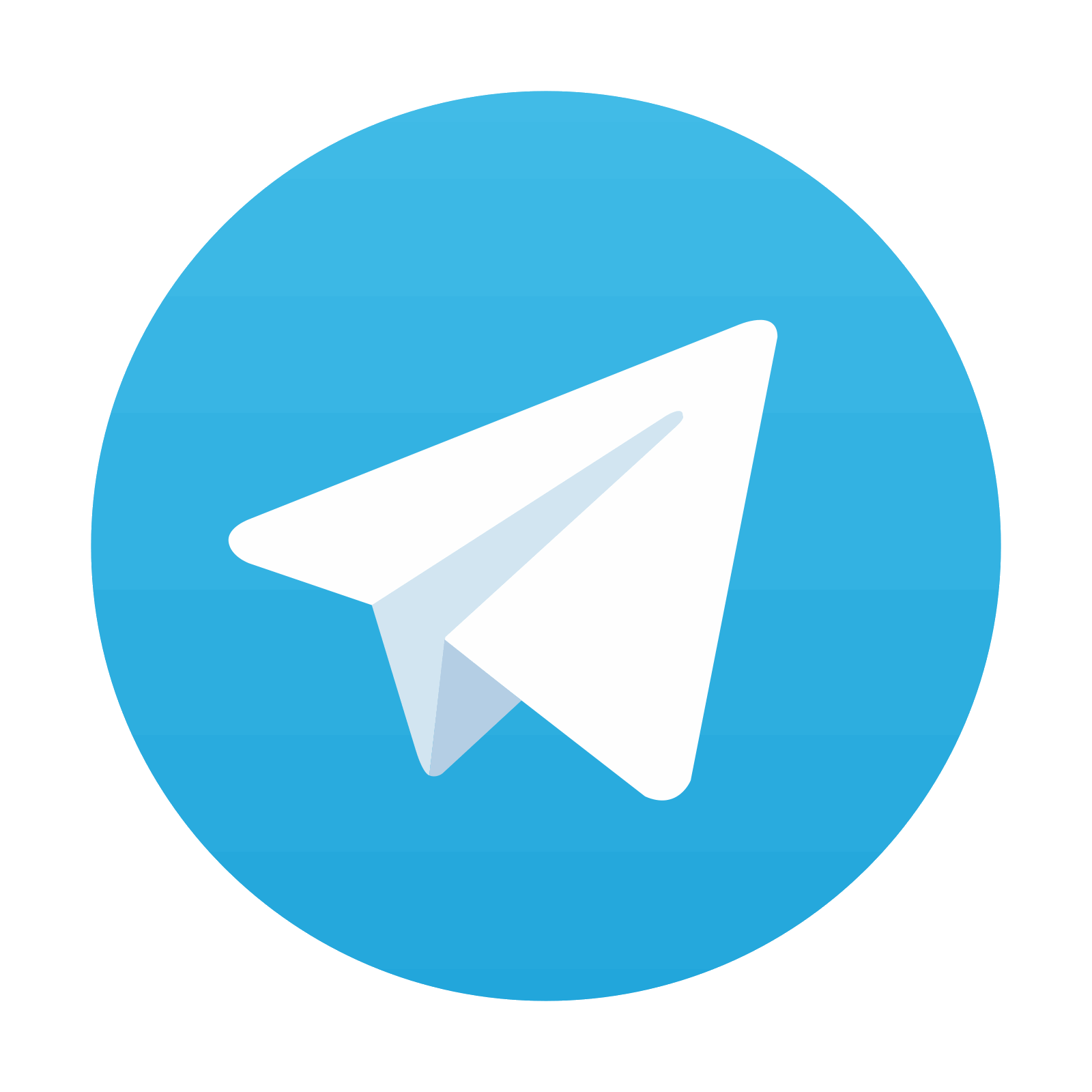
Stay updated, free articles. Join our Telegram channel
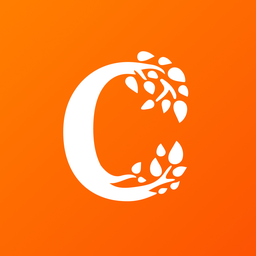
Full access? Get Clinical Tree
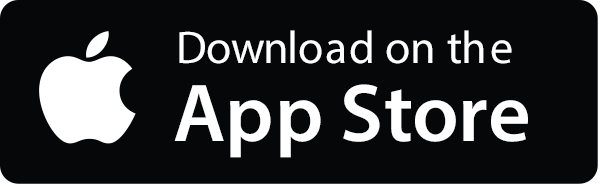
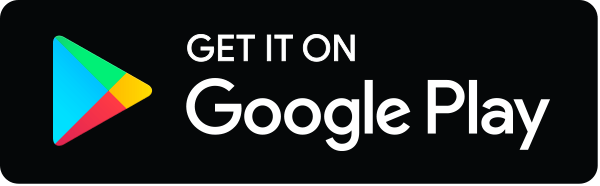